P. Kostka , R. Höhne , B. Maron , J. Ehlig , W. A. Hufenbach
Institute of Lightweight Engineering and Polymer Technology, TU Dresden, Dresden, 01307, Germany
Correspondence to: P. Kostka , Institute of Lightweight Engineering and Polymer Technology, TU Dresden, Dresden, 01307, Germany.
Email: |  |
Copyright © 2012 Scientific & Academic Publishing. All Rights Reserved.
Abstract
Textile-reinforced plastics are of continuously rising interest for industrial applications due to their tailorable properties and large-scale production capability. The utilisation of this novel material group as components in multi-material structures for complex lightweight applications with a high degree of function integration is focussed within the research of the collaborative research centre (SFB) 639. Lightweight design and function integration potentials of these novel structures are examined using an adaptive, self-diagnosing leaf spring within the framework of a demonstrator vehicle. In this article, a concept for the leaf spring design and the actuation mechanism based on an internal pressure cavity for the realisation of a stiffness adaptation are presented. A multidisciplinary analysis concept was established including three sub-models - an analytical based Fast Design Tool, a Finite Element Model and a Multi-body simulation model. The investigations include the simulation of the leaf spring characteristics for different internal pressures and the influence on the static and dynamic behaviour of the demonstrator vehicle.
Keywords:
Textile-reinforced Thermoplastics, Adaptive Composite Structure, Multidisciplinary Analysis
Cite this paper: P. Kostka , R. Höhne , B. Maron , J. Ehlig , W. A. Hufenbach , Multidisciplinary Analysis Concept for the Development of a Composite Adaptive Leaf Spring, International Journal of Composite Materials, Vol. 3 No. 6B, 2013, pp. 10-16. doi: 10.5923/s.cmaterials.201310.02.
1. Introduction
The outstanding properties such as high strength and stiffness in combination with a low specific mass and the numerous possibilities of function integration make the still recent material group of textile-reinforced thermoplastics interesting for future lightweight applications in industry[1]. Textile-reinforced thermoplastic composites can be tailored to structural demands and combined in multi-material applications in a much more flexible manner than conventional materials. To comply with the current demands of industry, new design methods for these complex highly integrative thermoplastic hybrid yarn structures are developed within the research of the collaborative research centre (SFB) 639. Especially functionalised composite structures with integrated sensor networks appear to be suitable for these demands. Therefore, innovative sensor networks for data acquisition, storage and transmission[2] as well as embedded actuators, which distribution and electrical connection is realised using special functional layers[3], are in the focus of the current research. New complex material models to describe the nonlinear material behaviour of the focussed glass fibre polypropylene (GF-PP) hybrid-yarn-textile-thermoplastic (HYTT) composites are developed[4]. In order to show the lightweight potentials of this novel material group, a prototypical application of all investigated technologies by means of a generic demonstrator vehicle “FiF” - a transport vehicle for goods to be conveyed e.g. for construction sites or factories - is developed within the project. Thereby, a special subcomponent is represented by the vehicle leaf spring, which demonstrates the integration of adaptive functions by means of an actuation mechanism, sensors networks as well as the application of developed material models and novel modelling strategies.
2. Physical Principal and Concept of Adaptive Leaf Spring
Within this research, lightweight design potentials of textile thermoplastic composites and new design methods are examined by developing an adaptive, self sensing textile-reinforced thermoplastic leaf spring within the framework of a demonstrator vehicle, Figure 1. One aim of the leaf spring development is to develop an actuating principle for varying the leaf spring stiffness, Figure 1. The adaption facilities self-levelling, body roll diminution in different situation, including cornering, accelerating and braking. Material-integrated sensors networks for measuring of loads and structural strain distribution deliver the input data for the control of these active functions. 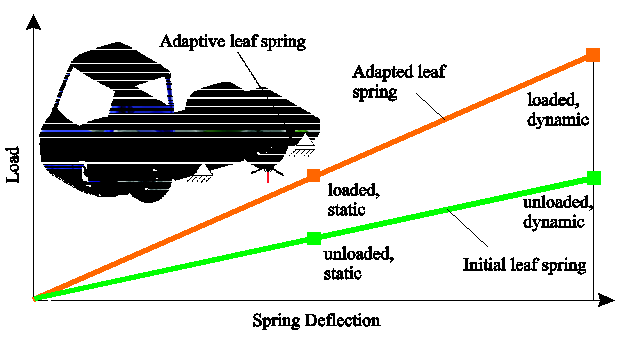 | Figure 1. Generic demonstrator vehicle “FiF” and idealised load-deflection diagram for an adaptive leaf spring |
To realise the adaptive function, a leaf spring system was developed, which can adjust its cross sectional area and thus its moment of inertia. In the developed solution, this is realised by means of a fluid filled cavity. While changing the internal fluid pressure or volume within the active area of the leaf spring, the height can be selectively adjusted, Figure 2.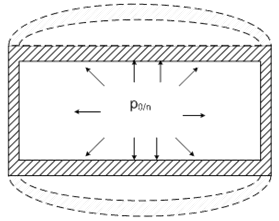 | Figure 2. Cross-section variation due to pressure generation in the leaf spring cavity |
The advantage of this adaptive system is the integrated solution without additional complex mechanical parts and the low need of energy during vehicle operation. Because, closing the fluid system the pressure can be kept constant. Additionally, as an alternative to the usage of e.g. hydraulic fluids, a particularly high degree of lightweight can be achieved by using air. Within this research framework intensive investigations of possible adaptive leaf spring design were carried out. As a result, a leaf spring in multi-material design with aluminium fillets and HYTT-belts was selected to be the best solution to sustain the complex stresses, Figure 3. The composite belts are supposed to be made of multi-layered flat-knitted fabrics (MKF). A more detailed description of the MKF and the according manufacturing process can be found in[5]. A symmetric layup of[0/90//90/0]2S was selected.Furthermore, design concepts and methods are studied with the intention to realise actively controlled vehicle dynamics and self monitoring of composite components using a common sensor system.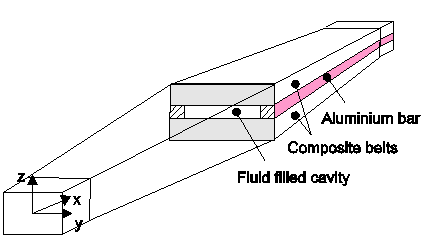 | Figure 3. Multi-material leaf spring design with global coordinate system |
3. Multidisciplinary Analysis Concept
A multidisciplinary analysis concept consisting of 3 submodels was established in order to enable a comprehensive, though efficient development of the adaptive leaf spring, Figure 4. Using the concept, the static and dynamic behaviour of the adaptive leaf spring and of the generic vehicle can be simulated. This enables the consideration of the manifold requirements for the leaf spring design at an early stage in the development phase.In this study, a fast design tool based on analytical formulation was developed in order to estimate a preliminary design that fits the given requirements. Subsequently, a finite element (FE)-model based on the geometry resulting from the preliminary design was developed. The FE-model is used in order to accurately compute the mechanical strains as well as the spring characteristic for different internal cavity pressures. A multi-body simulation (MBS)-model of the whole vehicle was developed, whereas the calculated stiffnesses and spring characteristics are used as model parameters. The MBS-model simulates the static and dynamic behaviour of the “FiF” demonstrator for the given configuration. Based on the numerical results, the design of the composite leaf spring can be optimised using an iterative procedure including the FE-model and the MBS-model. 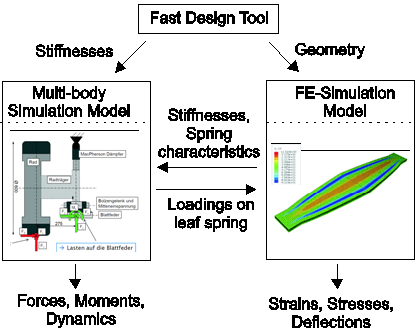 | Figure 4. Multidisciplinary Analysis Concept and Data Flow |
4. Fast Design Tool
Due to the very complex loading conditions, the design of the aspired inflatable composite structure is challenging. For the design identification, a realistic simulation using numerical methods is too extensive and time consuming. For that reason, analytical methods are used to describe the focussed problem. A fast design tool is proposed based on analytical formulas and an optimisation algorithm is used in order to identify the design that fits a specified objective. The identified preliminary design was used further for detailed analysis by means of the FE-Model.
4.1. Analytical Formulation
On the basis of the geometry and the loading conditions of the focussed adaptive leaf spring, two basic 2D-models were established for the derivation of the analytical formulas, Figure 5. The models represent the basic loading of the spring; namely the deflection due to a vertical load and the inflation due to a pressure inside the cavity. Since both loadings interact, the formulas of the 2D-models need to be combined in order to estimate the stresses and the deflection of the adaptive leaf spring at last.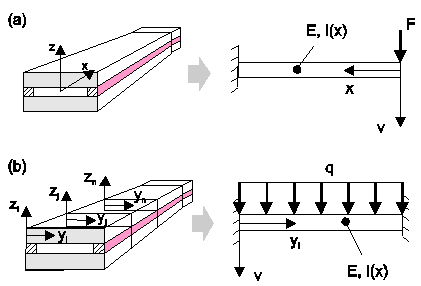 | Figure 5. Analytical cantilever beam model (a) and double cantilever beam model (b) representing different sections of the adaptive leaf spring |
The first model describes half of the leaf spring along its longitudinal direction as a cantilever beam with initially hollow-shaped rectangular section, Figure 5 (a). In the model the symmetry of the leaf spring supported on both sides is exploited. A vertical point force is applied at the free end of the cantilever beam, which causes shear stress and bending stress in the x-z plane. Due to the hollow shaped section, the shear is transferred by the relatively thick bars and thus can not be neglected. The maximum shear stress in the bars was approximated by[6] 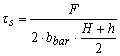 | (1) |
where F denotes the applied vertical force, bbar is the width of the aluminium bar, H and h are the height of the leaf spring and the cavity, respectively. The maximum bending stress σb at an arbitrary beam section in longitudinal direction can be expressed in the local coordinate system as 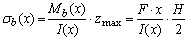 | (2) |
where Mb is the bending moment due to the point load, I is the moment of inertia of the corresponding cross section and
is the maximum distance to the neutral axis. The maximum bending stress may not exceed the bending strength of the respective material. The deflection of the cantilever beam is given by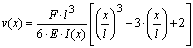 | (3) |
The second model describes the belt of the leaf spring at an arbitrary longitudinal position along its transversal direction as a double cantilever beam, Figure 5 (b). Bend resistant corners and no deformation of the bars are assumed. In the model, the pressure is approximated as a constant line load | (4) |
with p as the pressure load and bref as an arbitrary reference beam width. The length of the idealised beam depends on the considered cross section of the leaf spring. The maximum bending stress σb in the local y-z plane at an arbitrary longitudinal position x can than be expressed as 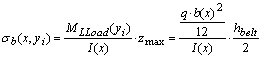 | (5) |
where MLLoad is the maximum bending moment due to the line load, I is the constant moment of inertia of the reference beam section, b is the width of the cavity and hBelt is the height of the belt. The deflection of the double cantilever beam is given as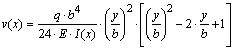 | (6) |
Since the belts deflect due to the inner pressure according to (6), the moment of inertia of the initial rectangular hollow-shaped section of the leaf spring changes. In order to calculate the stress and deflection due to the vertical load, the actual moment of inertia needs to be calculated and inserted in (2) and (3).Considering the stress state in the belts, the bending stress due to the vertical point load in the x-z plane and the bending stress due to the pressure load in the y-z plane are superimposed. As a result, a biaxial stress state in the belts occurs, stressing the layers of the crossply lamina parallel and transverse to the fibre direction.
4.2. Design Identification
In general, the optimal design of an adaptive leaf spring for lightweight applications features a maximum stiffness adaption and a minimised weight under consideration of the prescribed material strength and available space. A well known principle to achieve a lightweight structure is to fully utilise the material strength throughout the supporting structure. This principle is adopted for the design identification taking the interaction of the combined loadings, by means of the given equations (1) to (6), into account. In order to predict failure in the composite belts of the adaptive leaf spring, a physically based Failure Mode Concept (FMC) according to Cuntze[7] was used. The FMC distinguishes between five failure modes, representing one theoretically independent failure mechanism in multidirectional laminates. The main advantage of the FMC is the strict allocation of a strength criterion to one failure mode and to one associated basic strength. For each failure mode, a mode reserve factor can be calculated, describing the material stain. In this study, the fibre tensile failure and matrix tensile failure are relevant due to the biaxial stress state in the belts. The corresponding mode reserve factors can be expressed as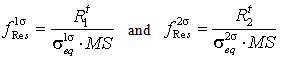 | (7) |
where
and
are the tensile strengths,
,
are the load induced stresses parallel and transverse to the fibre direction and MS is the margin of safety set to 2. The (resultant) Reserve Factor
takes the interaction of all failure modes into account by rounding-off the two mode reserve factors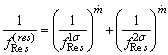 | (8) |
with
as the rounding-off exponent, set to a constant value of 6. The material fails, if one of the reserve mode factors or the resultant reserve factor is smaller than or equal to one. According to the specified design principle, the corresponding objective function is formulated as  | (9) |
The complete specification of the design identification problem is summarised in Table 1. Table 1. Problem specification of the design identification 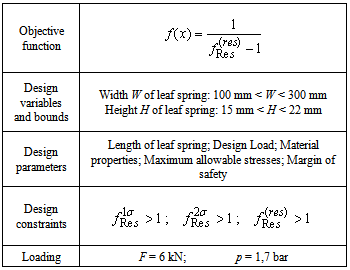 |
| |
|
The Generalized Reduced Gradient Algorithm is used in order to solve the nonlinear identification problem. Totally, seven equally-spaced crosssections were evaluated for the design identification. The identified preliminary design for the identified adaptive leaf spring is shown in Figure 6.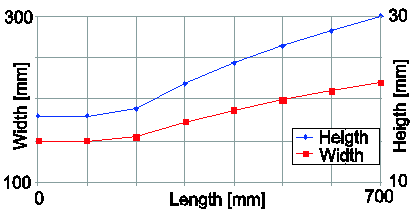 | Figure 6. Geometry of the identified, preliminary adaptive leaf spring design |
5. Finite Element Simulation
The pre-identified leaf spring design was modelled using Abaqus (version11.2). The FEmodel can be used for extensive studies regarding the optimal design identification, taking the complex 3d-stress states as well as the nonlinear geometrical and material effects into account. In order to provide basic data for the multi-body simulation, the determination of the spring characteristic of the pre-identified leaf spring was focussed first. The symmetrical modelling technique was used in order to reduce the computational efforts significantly. The existing geometry, material properties as well as the boundary conditions and the loading allows modelling only a fourth of the leaf spring, Figure 7. The finite element mesh consists of approx. 12000 cubic elements with linear displacement approach (Abaqus element type C3D8I), which feature an improved representation of the shear behaviour compared to standard cubic elements[8]. In order to avoid rigid body movements, displacement constraints were defined. Furthermore, the connection between the metal bars and the composite belts is assumed as ideal and remains unconsidered. For the further simplification of the modelling, a linear material model is used with material parameters experimentally determined within the SFB 639 subprojects C1 and C4, Table 2. 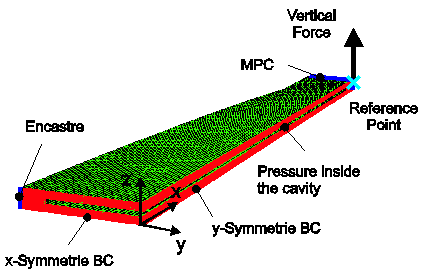 | Figure 7. FE-Model of the preliminary adaptive leaf spring design |
Table 2. Experimentally determined material parameters of HYTT ply 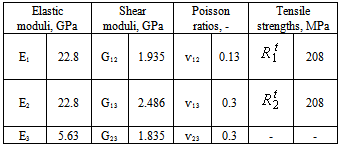 |
| |
|
A reliable simulation of the spring characteristic requires the consideration of the coupling effects of the fluid filled cavity with the surrounding composite structure. In order to represent the hydrostatic fluid and to simulate the fluid-structure interaction, hydrostatic fluid elements provided in Abaqus were used. With these elements, the fluid-structure interaction assuming either constant pressure or constant volume of the fluid can be modelled[9]. In this study, the characteristics of a leaf spring with a fully enclosed cavity filled with oil, whereas the volume of the oil remains constant, are simulated. The simulation was performed for several inner pressures up to 1.0 bar and a vertical load of 6 kN, which is applied via Multiple-Point-Constraints (MPC) to the structure. The deflection and the applied point load were extracted at the reference point and the spring characteristics were retrieved finally, Figure 8. The results illustrate the stiffness adaptation of the leaf spring due to the expanding cross-section of the leaf spring. The progressive curvature is due to buckling of the belts, once a critical load is exceeded. This problem needs to be addressed in following studies, for example through the integration of local stiffeners. Aside from that, the adaptability of the analytical Fast Design Tool is justified, since the occurring stresses are within the allowable range. For further analysis purposes, the spring characteristics and the leaf spring dimensions are transferred to the multi-body simulation software. 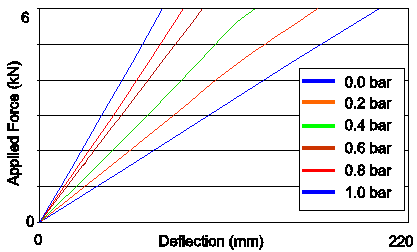 | Figure 8. Numerical calculated Load-Deflection Curve of adaptive leaf spring for different inner pressures |
6. Multi-body Simulation
The multi-body simulation model (build with SimulationX version 3.5) of the vehicle “FiF” presented in[9], was extended by two leaf springs with adaptive stiffness and with additional single-wheel suspension details. The leaf springs are part of the rear suspension system and were modelled by a predefined 3D-spring model provided by SimulationX. Their location as a part of the rear suspension kinematics and the vehicle coordinate system is illustrated by the 2D-view of the 3D-model in Figure 9 (a). The simplified suspension kinematics are shown in Figure 9 (b). 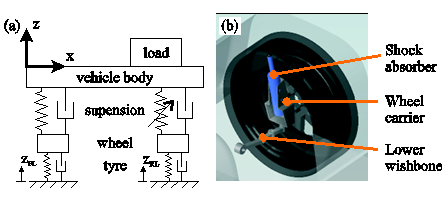 | Figure 9. (a) Simplified multi-body model, (b) Front wheel suspension |
To simulate driving on an arbitrary road, the whole system is excited through a translative preset in z-direction at each tyre. The road is described by a discrete time diagram depending on its quality and the vehicle velocity. Examples for a subjectively good road (i.e. urban street) and a bad road (i.e. construction site), referring to ISO 8608: 1995 (E), are shown in Figure 10.Especially for transport vehicles like the “FiF”, besides the riding comfort the stability and safety of the transported load is of major interest. Hereby, it is of particular interest to lower the acceleration amplitudes of the load platform and to equalize an unbalanced load. It is well known that a softer suspension reduces the acceleration amplitudes of the vehicle body and the dynamic wheel load within the range of the eigenfrequency of the vehicle body[10]. In the range of wheel frequency a tight suspension leads to lower dynamic wheel forces. In order to balance out the load platform horizontally, the pressure of the leaf springs can be adjusted separately. For detecting an inclined position, a simple position sensor can be used. The more difficult task is to determine the unevenness of the road the vehicle is driving on. For the multi-body simulations, it was not taken into account, that such a proper detecting system is needed. 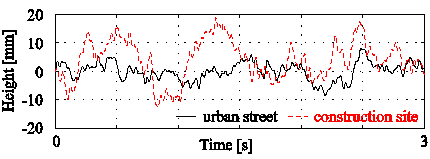 | Figure 10. Random road profiles with different quality |
A test drive on the generated construction site course comparing three scenarios was simulated: Scenario 1: no loading and no inner pressureScenario 2: 200 kg loading and no inner pressureScenario 3: 200 kg loading and 0.2 bar inner pressureFigure 11 shows the results of the spring deflection of the rear right leaf spring, the vertical load at the rear right wheel and the vertical displacement of the load platform for a time period of 1s for all scenarios.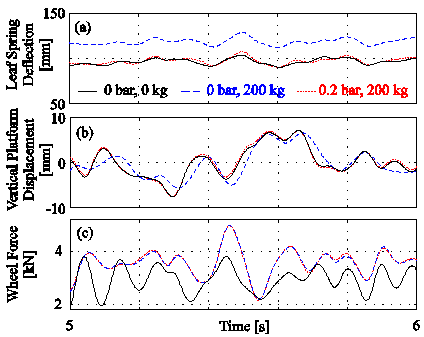 | Figure 11. Discrete time results of a simulated drive on a construction site |
The mean spring deflection of 96 mm rises up to 118 mm due to the applied load. If the pressure is raised to 0.2 bar, the mean deflection has its former magnitude. The effect of raising the pressure by 0.2 bar on the dynamic wheel load is minimal. Comparing the vertical travel of the load platform of the initial scenario with the loaded vehicle with adapted leaf spring stiffness, just slight deviations can be identified. Another simulation task dealt with an additional load of 300 kg that was placed on the right side of the vehicle. The system was excited two times with the profile of an urban street and a velocity of 65 km/h, once without stiffness adaption (both leaf springs with 0.4 bar) and once with an inner pressure of 0.8 bar for the right leaf spring. The maximal absolute rotation angle of the vehicle body around the x-axis was reduced by 15%, Figure 12. 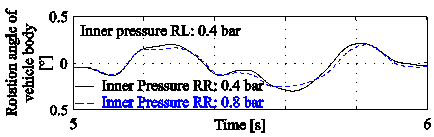 | Figure 12. Influence of different spring stiffnesses at rear right (RR) and rear left (RL) wheel on rotation angle around x-axis of vehicle body due to unbalanced load |
A future simulation task should imply the automatic setting of the correct pressure due to different load and road conditions through extending the model by a control loop. Here, it is necessary to distinguish between fast and slow control needs. For horizontal balancing of the load platform, a slow control loop is needed which is not reacting to the dynamic wheel travel which is caused by the unevenness of the road.
7. Conclusions
The potentials of textile-fibre reinforced thermoplastics as components in an adaptive leaf spring made in multi-material design are investigated numerically. In order to realise the adaptation of the spring characteristic, an actuation mechanism by means of a fluid filled cavity inside the leaf spring was proposed. A multidisciplinary analysis concept was established, which allows the consideration of the manifold requirements, e.g. the static behaviour of the leaf spring and the dynamic behaviour of the vehicle, at an early stage of the development phase. According to the concept, a preliminary design of the adaptive leaf spring was identified by means of an analytical Fast Design Tool. Subsequently, a FE-simulation confirmed the functionality of the proposed actuation mechanism by means of the leaf spring adaptation capabilities. A multi-body simulation demonstrated that for static applications, e.g. levelling the platform, the adaptive leaf spring considerably influences the vehicles behaviour. However, the effect on the dynamic behaviour of the vehicle is limited. In order to extend the controlled stiffness range of the leaf spring, an optimal design identification using an iterative procedure based on the established multidisciplinary analysis concept is planned. Therefore, previously identified nonlinear material behaviour of the HYTT will be considered. In order to experimentally validate the used concepts and models, the manufacturing of the identified leaf spring and basic tests are intended.
ACKNOWLEDGEMENTS
The authors would like to express their gratitude towards the Deutsche Forschungsgemeinschaft (DFG), for funding the research in the scope of the subprojects E1 and C1 of the Collaborative Research Centre SFB 639 “Textile-Reinforced Composite Components in Function-Integration Multi-Material Design for Complex Lightweight Applications” and the whole research community of the SFB 639.
References
[1] | W. Hufenbach, “Textile Verbundbauweisen und Fertigungstechnologien für Leichtbaustrukturen des Maschinen- und Fahrzeugbaus”, SDV - Die Medien AG, 2007. |
[2] | K. U. Roscher, W. J. Fischer, J. Landgraf, G. Pfeifer, E. Starke, “Sensor networks for integration intotextile-reinforced composites”, The 14th international conference on solid-state sensors, actuators and microsystems, Lyon, France, 2007. |
[3] | W. Hufenbach, P. Kostka, K. Holeczek, A. Filippatos, “Monitoring of a composite plate using integrated vibration measurement system”, International Symposium on Piezocomposite Applications, ISPA 2011, Dresden, Germany. |
[4] | R. Böhm, M. Gude, W. A. Hufenbach, “Phenomenologically based damage model for 2D and 3D textile composites with non-crimp reinforcement”, Materials and Design 32, p. 2532-2544, 2011. |
[5] | W. Hufenbach, N. Petrinic, A. Hornig, A. Langkamp, M. Gude, J. Wiegand, „Delamination behaviour of 3D-textile reinforced composites – experimental and numerical approaches“, The e-Journal of Nondestructive Testing, 11 (12), 1st Conference on damage in composite materials, Stuttgart, Germany, 2006. |
[6] | W. Müller and F. Ferber: “Technische Mechanik für Ingenieure”, Carl-Hanser-Verlag, 2008. |
[7] | R.G. Cuntze and A. Freund, “The predictive capability of failure mode concept-based strength criteria for multidirectional laminates” Composites Science and Technology, 64 (2004), 343-377. |
[8] | Dassault Systems, Abaqus 6.11 Online Documentation, 2012. |
[9] | W. A. Hufenbach, P. Kostka, B. Maron, D. Weck, J. Ehlig, M. Gude, M. Zscheyge, “Development and investigation of a textile-reinforced thermoplastic leaf spring with integrated sensor networks”, Materials Science Engineering Symposium B6 – Hybrid Structures. |
[10] | E. Göhring, R. Povel, E.-C. von Glasner, P. Schützner, "Semiaktiv und aktiv geregelte Luftfedersysteme für Nutzfahrzeuge", VDI-Berichte Nr. 916, 1991. |