Shantanu Srivastava 1, Mrinal Kaushik 2
1Department of Mechanical Engineering, Pranveer Singh Institute of Technology, Kanpur, India
2Department of Aerospace Engineering, Indian Institute of Technology, Kharagpur, India
Correspondence to: Mrinal Kaushik , Department of Aerospace Engineering, Indian Institute of Technology, Kharagpur, India.
Email: |  |
Copyright © 2015 Scientific & Academic Publishing. All Rights Reserved.
Abstract
The jet mixing efficiency of a circular cross-wire, running along the diameter, kept at the exit of a Mach 1.8 square nozzle, was studied at varied levels of expansion present at the nozzle exit. The experimental investigations were conducted at nozzle pressure ratios (NPRs, defined as the ratio of settling chamber pressure to the back pressure) of 5.5, 6.0 and 6.5. The centerline pressure decay and the pressure profiles were plotted, for both uncontrolled and controlled jets. The waves present in supersonic jet core were captured using Shadowgraphic flow visualization method. A maximum of 88% reduction in the supersonic core length was obtained at NPR 6, for the jet controlled with circular cross-wire.
Keywords:
Free jets, Shock-Cells, Mixing augmentation, Pressure profiles, Flow visualization
Cite this paper: Shantanu Srivastava , Mrinal Kaushik , Supersonic Square Jet Mixing in Presence of Cross-Wire at Nozzle Exit, American Journal of Fluid Dynamics, Vol. 5 No. 3A, 2015, pp. 19-23. doi: 10.5923/s.ajfd.201501.03.
1. Introduction
It is well known that, the optimum proportion of large and small scale vortices are effective in promoting mixing [1]. From the vortex theory, it is also understood that the size of vortices are governed by the radius of curvature from which they are shed [2]. One such method to the shed the vortices of mixed size is using non-circular nozzle exit geometries such as elliptical, triangular, square, and rectangular. It is due to their varying azimuth which shed the vortices in different combinations. A quantum of work is carried out by many researchers in the past using non-circular nozzle exit geometries, exhibiting augmented mixing. Rectangular and triangular nozzles have flat sides and corners, which are beneficial for combining large scale mixing at the flat sides with small scale mixing due to corner flow [3, 4]. Because the spreading rate at the flat sides is larger than at the corners, therefore, axis-switching takes place. The small radius of curvature at the corners induces vortex deformation, which eventually evolve into streamwise vortices. The ensuing complex vortex interaction results in improved large and small scale mixing. Supersonic combustion tests conducted with these nozzles increased the combustion heat release. Further, the coexistence of regions with large scale structures and others with fine scale structures in the same flow field of triangular and square jets is advantageous for combustion systems. At the flat sections of the shear flow, the large scale mixing provides the necessary amounts of air to sustain the combustion. The enhanced fine scale mixing at the corners makes this section to be the best location for fuel injection. The highly turbulent flow at the corners helps to start the reaction closer to the nozzle exit. Also, the periodic heat release associated with the reaction process (which occurs in the vortices cores) is reduced. This eliminates the excitation source of combustion oscillations. This concept was studied by Gutmark et al., (1989) [5], as a possible passive control method to reduce combustion instabilities in the operation of dump combustors. Quinn, (1992) [6], shed some light on the streamwise development of the square jet cross section. It was reported that the near region of the jet is dominated by four sets of counter rotating streamwise vortices. These vortices are generated from the distributed vorticity shed by the four corners of the nozzle by skewing of shear layers as a result of vena-contracta effect.With the above results, it can be observed that all these researchers found enhanced fine scale mixing with the introduction of sharp corners into the jet. In the jet literature, it is also well established that in addition to using non-circular geometries, the mixing can be augmented further if the vortices are shed closer to the jet centerline. Sreejith and Rathakrishnan (2002) [7], used a wire running across a diameter (cross-wire) as a passive control to enhance the jet mixing. The streamwise vortices introduced by the cross-wire lead to a more rapid decay of the centerline Pitot pressure. Also, the cross-wire was found to weaken the shock-cells in the jet core significantly. This passive control found to be effective at all levels of expansion. However, in this study the nozzle exit cross-section was circular. Wang et al., [8], studied the near field of the square jet with vortex generating delta tabs. They used four vortex generating tabs and concluded that the arrangement had an effect of suppressing the occurrences of axis-switching. In the study carried out by Rathakrishnan (2009) [9], it was found that the cross-wire performed effectively in promoting the jet mixing near to correct-expansion.In the view of aforesaid discussion on cross-wire as non-circular jet control, it was almost inevitable to investigate again the optimized combination of non-circular nozzle exit geometry and levels of expansion, in promoting the jet mixing. With this motivation, a circular cross-wire running along the diameter of a Mach 1.8 square convergent-divergent nozzle, under marginally overexpanded, almost correctly-expanded, and marginally underexpanded states of the jet has been experimentally investigated in the present study.
2. Experimental Details
In the present study, a convergent-divergent Mach 1.8 nozzle was experimentally investigated. A brass made cross-wire of 0.5 mm in diameter, was used as jet control. In order to keep the thrust loss to a minimum because of the flow hindrance caused by the cross-wire, the projected area of the cross-wire (normal to jet exit), was kept below 5% of the nozzle exit area. The schematic diagram of square nozzle controlled with cross-wire is shown in Figure 1.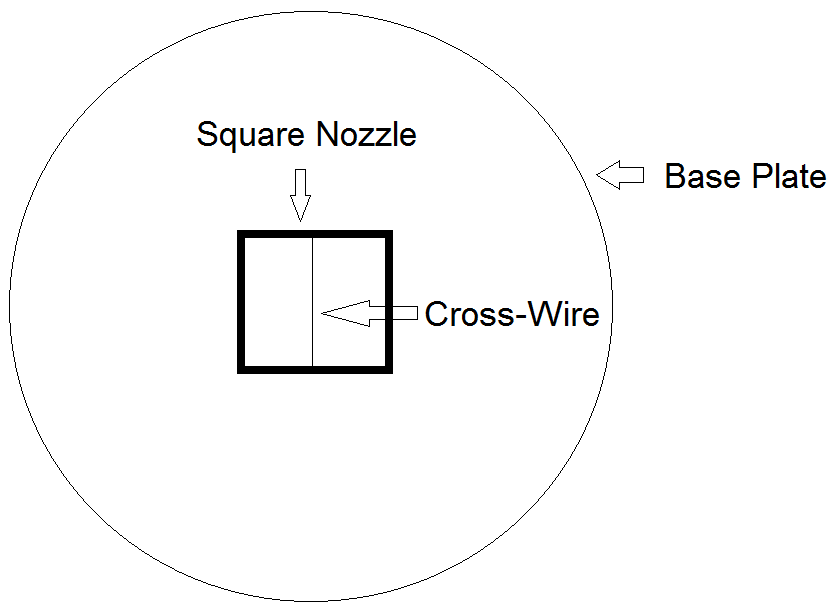 | Figure 1. Schematic diagram of Mach 1.8 square nozzle with a cross-wire at the exit |
Pitot pressures were measured using a PSI 9016 pressure transducer. The transducer had 16 pressure ports with the capability of measuring pressures simultaneously through all the ports using a menu driven user-friendly software provided by the manufacturer. The pressure measuring accuracy of the transducer (after re-zero calibration) was specified to be ±0.15% on a full scale. The pressure measurements were conducted using a Pitot probe mounted on a 3D-traverse with the specified least count of 1 mm in translation. The probe mounted on the traverse measured the pressures along the jet axis (X-axis), in the direction normal to the tab (Y-axis), and along the tab (Z-axis), at 1 mm intervals, with the origin at the centre of the nozzle exit plane (Figure 2). 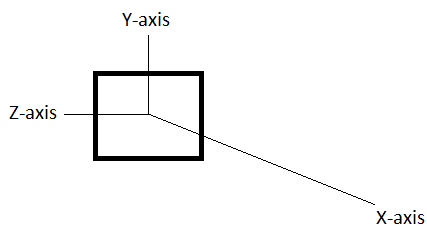 | Figure 2. Schematic diagram of co-ordinate axes |
The effectiveness of jet controls in weakening the shock cells were qualitatively analyzed using Shadowgraphic optical flow visualization technique.
3. Results and Discussion
It is well established that supersonic jet core is a highly wave dominated zone and precise calculation of Mach numbers at every location from the measured Pitot pressures, is a cumbersome task [10]. This is due to the unavailability of a single closed-form analytical relation, which is valid for all the waves present in the supersonic jet core. Hence, in order to quantify the jet mixing, the measured Pitot pressures
have been non-dimensionalized with the settling chamber pressure
and plotted against the non-dimensional axes distances (X, Y, and Z) traversed by the probe. The axes distances have been made non-dimensional with the equivalent diameter (D) of the square nozzle exit area.
3.1. Centerline Pitot Pressure Decay
The centerline pressure decay for both uncontrolled and controlled jets, at marginally overexpanded condition corresponding to NPR 5.5, is presented in Figure 3. In case of uncontrolled jet, there exist 6 prominent shock-cell structures with waves of considerable strength. The supersonic jet core length extends up to about X/D = 8.6. However, when the cross-wire is introduced at the nozzle exit the core length is drastically reduced to 1.5D. That is, about 83% reduction in core length is obtained by using cross-wire at the nozzle exit. Hence, it is explicit that, the mixing promoting small scale vortices introduced into the jet field for the controlled jet are able to promote the jet mixing to a very great extent. It is also observed that beyond X/D = 25, both uncontrolled and controlled jets are in fully developed states. 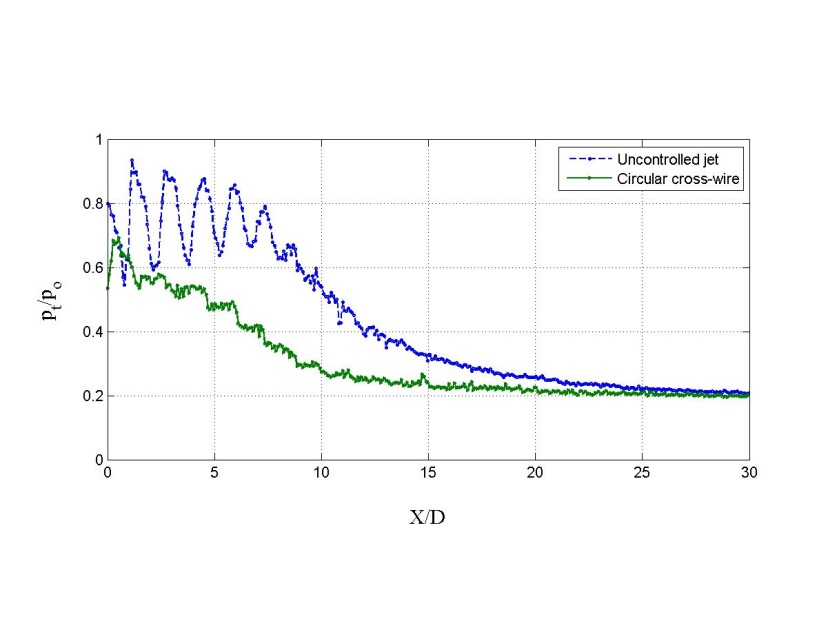 | Figure 3. Jet centerline pressure decay of Mach 1.8 at NPR 5.5 |
At NPR 6, which is almost correctly-expanded state for Mach 1.8 jet, the centerline pressure decay results are shown in Figure 4. It is found that, for this NPR the uncontrolled jet core extends up to about X/D = 10.6, whereas, for controlled jet the core length is found to be just X = 1.3D. Also, the characteristic decay for the controlled jet at zero pressure gradient is found to be steeper than the NPR 5.5 case. Further, at this NPR also beyond X = 25D, the far field jet mixing in both control and uncontrolled jets approach each other. Thus, in the presence of almost zero-pressure gradient, the cross-wire could able to promote the jet mixing significantly, resulting in a core length reduction of 88%. 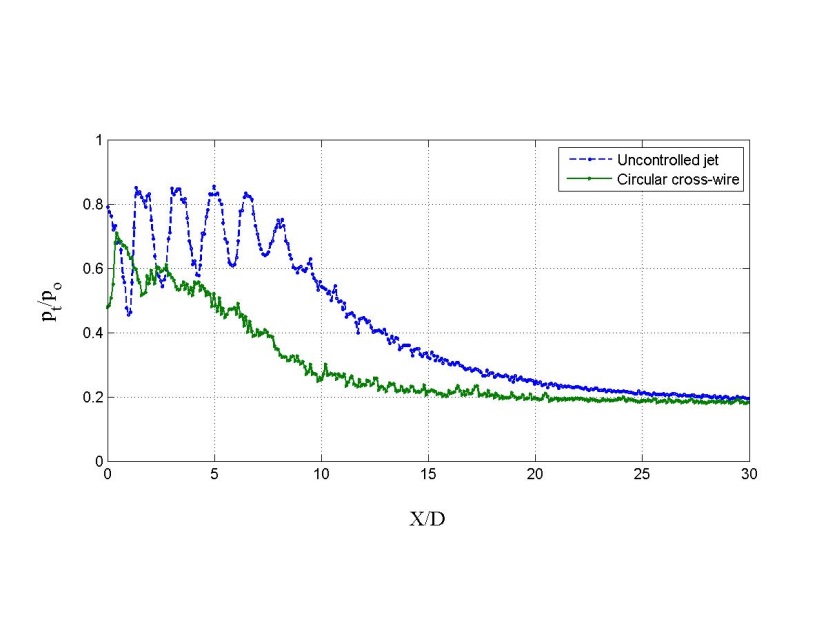 | Figure 4. Jet centerline pressure decay of Mach 1.8 at NPR 6.0 |
At marginally underexpanded state corresponding to NPR 6.5, the results are shown in Figure 5. Again in this case also, the supersonic core length for the uncontrolled jet is found to be 11.7D, whereas it is just 1.6D for the controlled jet. That is, as high as 85% reduction in core length is achieved for the controlled jet with favorable pressure gradient present at the nozzle exit. 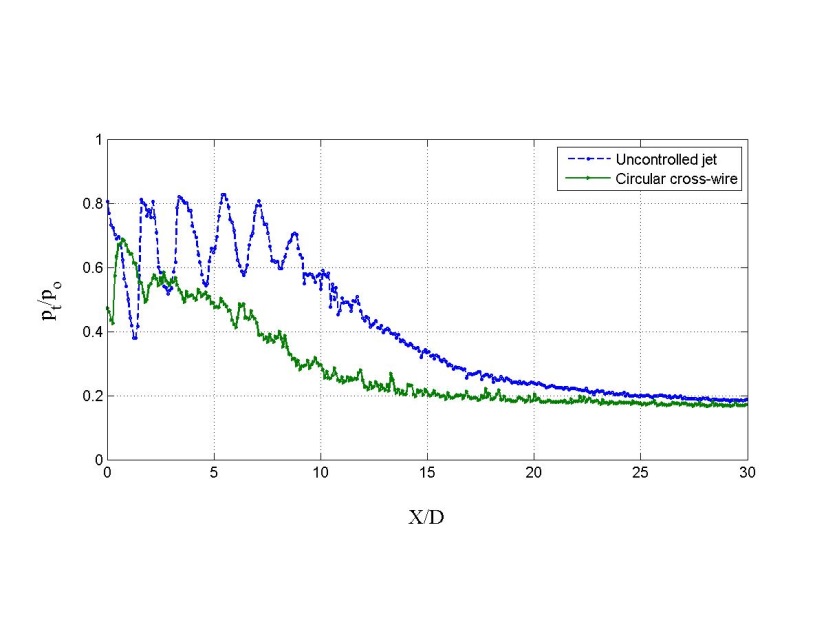 | Figure 5. Jet centerline pressure decay of Mach 1.8 at NPR 6.5 |
Hence, the centerline pressure decay results demonstrate that, the mixing promoting efficiency of a cross-wire kept at the exit of a Mach 1.8 square nozzle is found to be the best near to correct-expansion. That is, the maximum core length reduction achieved is about 88% at NPR 6, for the controlled jet. This result is considerably higher in terms of core length reductions as compared to the 50% reduction obtained in circular nozzle controlled with cross-wire [9].The higher mixing achieved in case of square nozzle over its circular counterpart may be due to the additional four sharp corners present in case of square nozzle. At each sharp corner, two opposite families of vortices are shed which might be interacting among themselves and hence inducing intense mixing in the jet field right from the nozzle exit. This additional mixing promotion caused by the sharp corners might be a primary cause for the greatly enhanced mixing experienced by a square jet compared to a circular jet under identical conditions.
3.2. Pressure Profiles
The non-dimensional pressure distribution at NPR 6, measured in two directions; along and normal to the cross-wire, are plotted for both uncontrolled and controlled jets (Figures 6-7).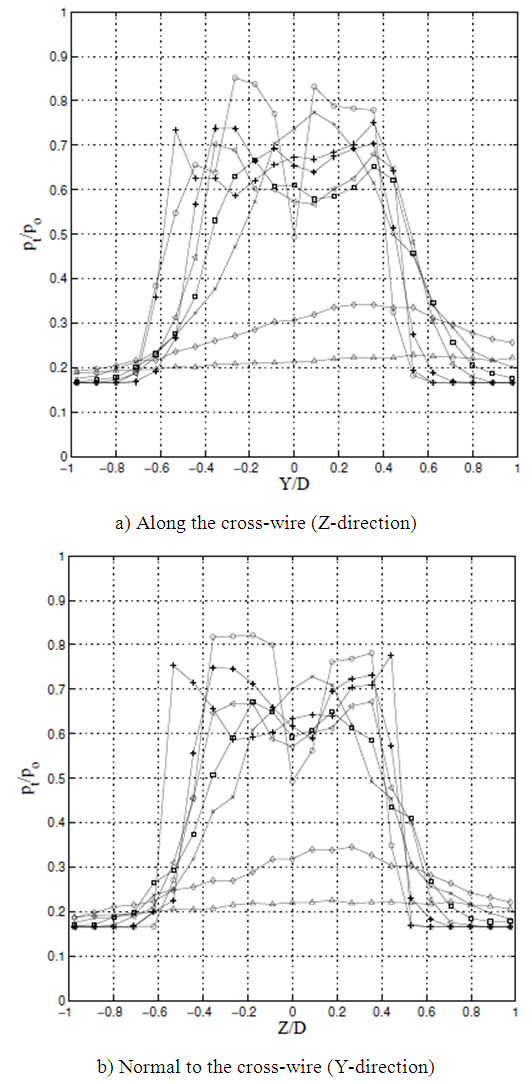 | Figure 6. Pressure profiles of uncontrolled jet at NPR 6 |
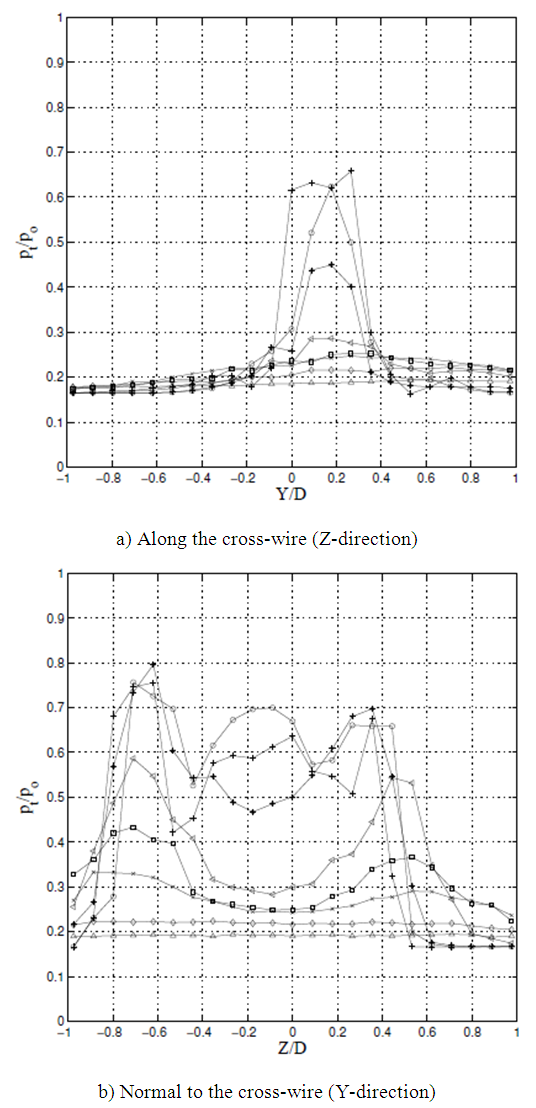 | Figure 7. Pressure profiles of controlled jet at NPR 6 |
The Pitot pressure profiles at different axial locations for the uncontrolled jet at NPR 6 are presented in Figure 6. It can be seen that the jet spread is increased in Z-direction compared to Y-direction. Also, the jet becomes asymmetric at some axial locations along the jet centerline. However, when the cross-wire is introduced at the nozzle exit the jet spread rate is considerably higher in Y-direction compared to Z-direction (Figure 7). The differential spread rate in the two different directions produces shear leading to an ‘axis-switch’, which is a direct measure of enhanced jet mixing. Further, it can also be observed that the cross-wire insertion is not introducing any appreciable asymmetry into the jet and the jet begins to bifurcate in the far-field.
4. Optical Flow Visualization
The Shadowgraphic optical flow visualization method was employed to analyze the waves prevailing in the jet with and without control at different NPRs. For control jets, visualization was carried out viewing along and normal to cross-wire, directions.The visualization image for the uncontrolled jet at NPR 6 is shown in Figure 8(a). The oblique shocks originating at the nozzle exit cross each other at the jet axis and meets the barrel shock at the jet periphery. Beyond this point, the oblique shock waves get reflected as expansion fan, because the reflection from free boundary is unlike. The `kink' formed at the shock cross-over points (along the jet axis) are, also seen in the Figure 8(a). Further, the expansion fans cross each other and reach the jet boundary and reflect back as compression waves. The reflected compression wave extends up to jet boundary and cycle repeats leading to a shock-cell structure. The distance between successive shock reflection points (`kinks') is called a shock-cell length.There are six visible shock-cells in the visualization image for the uncontrolled jet (Figure 8(a)). Further, it is interesting to see the pattern of oblique shocks at the nozzle exit. Though, the first shock cell is longer however, the shock-cells following the first shock-cell are weaker and shorter. This might be due to the enhanced mixing caused by the vortices shed from the sharp corners of the nozzle with square cross-section from inlet to exit. The visualization images of the controlled jet at marginally overexpanded state corresponding to NPR 6, are shown in Figures 8(b)-(c). It is seen that though a number of prominent shock-cells are still visible in Y-direction but the shock strength is considerably reduced (Figure 8(b)). Further, the bifurcation of jet in the proximity of nozzle exit is also clearly visible in direction, along the cross-wire (Z-direction), confirming the vortex shedding right at the nozzle exit, responsible of enhanced mixing (Figure 8(c)). The above results support the inferences obtained in centerline pressure decay and pressure profiles sections.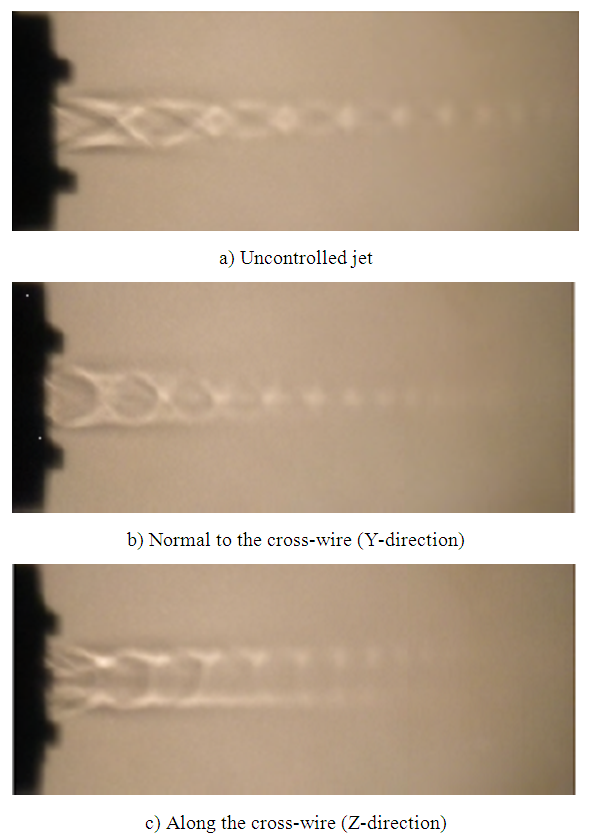 | Figure 8. Shadowgraphic images of Mach 1.8 jet at NPR 6 (overexpanded) |
5. Conclusions
The mixing enhancement efficacy of a cross-wire running along the diameter of a Mach 1.8 convergent-divergent nozzle, under varied levels of expansion present at the nozzle exit, has been experimentally investigated. It was found that the presence of cross-wire initiates the jet mixing early, leading to a maximum core length reduction of 88% at NPR 6. It was also evident that the cross-wire was capable of promoting jet mixing right from the nozzle exit. The pressure profiles confirmed that the cross-wire was effective in mixing enhancement without introducing any appreciable asymmetry in the jet. The visualization images revealed the attenuation of waves prevailing in the jet core, which could be the direct evidence of mixing augmentation. Also, for the controlled jet the shock-cells became shorter and waves became weak in nature.
ACKNOWLEDGEMENTS
The authors acknowledge the financial support provided by Sponsored Research and Industrial Consultancy (SRIC), Indian Institute of Technology Kharagpur in order to complete the present investigation. The authors also acknowledge the High Speed Aerodynamics Laboratory at Indian Institute of Technology Kanpur, where the experiments in the present study has been conducted.
Nomenclature
AR = Aspect ratio (ratio of semi-major axis to semi-minor axis lengths)D = Equivalent diameterNPR = Nozzle Pressure Ratio, 
= Pitot pressure
= Settling chamber pressure
= Pitot pressure in the jet fieldX = Coordinate along the jet axisY = Coordinate axis normal to cross-wire directionZ = Coordinate axis along the cross-wire direction
References
[1] | Krothapalli, A., Hsia, Y., Bagano. D., and Karamcheti, K., 1986, The Role of Screech Tones on Mixing of an Underexpanded Jet, Journal of Sound Vibration, Vol. 106, pp. 119–143. |
[2] | Houghton, E.L., and Carpenter, P.W., Aerodynamics for Engineering Students, Fifth Edition, Butterworth-Heinemann, 2003. |
[3] | Gutmark, E.J., Schadow, K.C., and Wilson, K.J., 1991, Effect of Convective Mach Number on Mixing of Coaxial Circular and Rectangular Jets, Physics of Fluids, Vol. 3, No. 1, pp. 29–36. |
[4] | Srinivasan, K., and Rathakrishnan, E., 2001, Studies on Underexpanded Rectangular Slot Jets, Journal of the Aeronautical Society of India, Vol. 53, No. 1, pp. 39–43. |
[5] | Gutmark, E.J., Schadow, K.C., Parr, T.P., Hansonparr D.M., and Wilson, K.J., 1989, Noncircular Jets in Combustion Systems, Experiments in Fluids, Vol. 7, pp. 248–258. |
[6] | Quinn, W.R., 1992, Streamwise Evolution of a Square Jet Cross Section, AIAA Journal, Vol. 30, No. 12, pp. 2852-2857. |
[7] | Sreejith, R.B., and Rathakrishnan, E., 2002, Cross-Wire as Passive Device for Supersonic Jet Control, AIAA Paper 2002–4052. |
[8] | Wang X.K., Chua L.P, and Yu. S.C.M., 2003, On the near-field of a square jet with vortex-generating tabs, Fluid Dynamics Research, Vol. 32, pp. 99-117. |
[9] | Rathakrishnan E., 2009, Experimental Studies on the Limiting Tab, AIAA Journal, Vol. 47, No. 10, pp. 2475-2485. |
[10] | Shapiro, A.H., Dynamics and Thermodynamics of Compressible Fluid Flow, John Wiley, USA, 1953. |