A. I. Oludare1, M. N. Agu2, A. M. Umar3, S. O. Adedayo4, O. E. Omolara5, L. N. Okafor6
1Nigerian Defence Academy, Department of Physics, Kaduna
2Nigeria Atomic Energy Commission, Abuja
3Energy Commission of Nigeria, Department of Nuclear Science & Technology, Abuja
4National Open University of Nigeria, Department of Information Technology, Abuja
5Ahmadu Bello University, Department of Mathematics, Zaria, Nigeria
6Nigerian Defence Academy, Department of Mathematics and Computer Science, Kaduna
Correspondence to: A. I. Oludare, Nigerian Defence Academy, Department of Physics, Kaduna.
Email: |  |
Copyright © 2012 Scientific & Academic Publishing. All Rights Reserved.
Abstract
The research conducted safety margin test on some typical or experimental design parameter dimensional change of graphite moderated reactor design (GMRD) models in terms of the height and diameter with respect to high temperature effect on the stability of the reactor during operation and secondly, safety margin test was conducted on the stability of the reactor during cooling problem of the fuel with respect to reactor core temperature using Linear Regression Analysis Techniques. This was achieved by the modification of the design parameter of the reactor graphite core height and diameter. The results of the statistical analysis on these types of nuclear reactor models reveals that the GMRD models promises stability under application of large size of graphite core at a height of 10.0metre and diameter of 3.5metre. Because at this parameter the temperature seems at maximum and the reactor agrees to be most stable as the regression plot was optimized, that is the least squares method finds its optimum when the sum, S, of squared residuals or errors are at minimum. Meanwhile, at anything below the height of 10.0metre and diameter of 3.5metre the fuel element seems to be unstable in the reactor as the regression plot could not find it optimum. The safety margin prediction of 0.95% was validated for a typical GMRD model as an advantage over the current 5.1% challenging problem for plant engineers to predict the safety margin limit.
Keywords:
Graphite moderated reactor, Reactor core design dimensional change, Large graphite core size, Height and diameter, High temperature effect, Fuel element, Reactor safety, Safety factor, Ỳ, Optimization, Stability margin, Graphite moderated reactor design models
Cite this paper: A. I. Oludare, M. N. Agu, A. M. Umar, S. O. Adedayo, O. E. Omolara, L. N. Okafor, High Temperature Effect on Graphite-Moderated Reactors Safety, Journal of Safety Engineering, Vol. 3 No. 1, 2014, pp. 1-12. doi: 10.5923/j.safety.20140301.01.
1. Introduction
Researches have shown that cooling problem of graphite moderated reactor especially during an accident can contribute to the acceleration of the reactor melt-down, since the design dimension of graphite height and diameter play significant role in the safety of these types of reactor[1]. This design dimension in terms of height and diameter of the reactor graphite core need to be considered in other to minimize high increase in the fuel temperature during operation and accident in other to avoid reactor melt-down and to keep the reactor safe. The large size of graphite core will provide low power density reactor that will minimize heat conservation in the reactor core and also disallow core melting. The small size of graphite core could contribute to the causes of decay heat within reactor core of nuclear power plant either during operation or accident. Graphite is the element that has the highest melting point (3675℃) that makes it desirable for reactor core than any other element. The decay heat in the core assemblies could degenerate to hydrogen built-up that can make reactor to fail, as identified in some reactor accidents[2]. Recently, data for the dimensional change of AGR graphite have been successfully fitted to irradiation temperature, ignoring any effect of fast neutron flux level (Eason e tal, 2006). In this work comparism of different test on graphite moderated reactor design (GMRD) models parameter specification of height and diameter was carried out before conclusion. Historically, “equivalent temperature” has been used to fit the temperature dependence of graphite dimensional change as a function of fast neutron irradiation. This paper purpose was to test fuel and materials. The reactor design concept is intended to allow for the high temperature effect on dimensional variation in the stability of commercial graphite moderated power reactors. It is hope that this conceptual design would provide a good, novel approach and method for multi-objective decision-making in the development of the nuclear industry when applied. We must know that the main drivers for reactor developments are:● Improved safety (for example by the incorporation of passive safety features)● Reduced capital cost● Reduced operating cost● Improved efficiency and utilization● Improved design effectiveness● Reduced build-time● Minimize the risk of failure and extrapolate the risk of failure
2. Graphite-Moderated Reactors
A graphite reactor is a nuclear reactor that uses carbon as a neutron moderator, which allows un-enriched uranium to be used as nuclear fuel. Nuclear graphite is any grade of graphite, usually electro-graphite, specificallymanufactured for use as a means of production (moderator or reflector) within nuclear reactors. Graphite is an important material for the construction of both historical and modern nuclear reactors as it is one of the purest materials manufactured at industrial scale and it retains its properties (including strength) even at high temperatures. There are several types of graphite-moderated nuclear reactors that have been used in commercial electricity generation these include:(i) High-temperature gas-cooled reactors (past) such as;■ Dragon reactor (used helium gas as coolant and coated particle fuel)■ The AVR reactor (German: Arbeitsgemeinschaft Versuchsreaktor)■ Peach Bottom Nuclear Generating Station, Unit 1(an experimental helium-cooled, graphite-moderated reactor)■ THTR-300 (a thorium high-temperature nuclear reactor)■ Fort St. Vrain Generating Station -is a natural gas powered electricity generating facility.(ii) High temperature gas-cooled reactors (in development or construction) such as;■ Pebble-bed reactor -( the design takes advantage of the inherent safety characteristics)■ Prismatic fuel reactor (uses TRISO fuel particles)■ UHTREX Ultra-high-temperature reactor experiment(iii) Water-cooled reactors such as;■ RBMK - (Russian: Реактор Большой Мощности Канальный Reaktor Bolshoy Moshchnosti Kanalnyy, "High Power Channel-type Reactor")(iv) Gas-cooled reactors such as; ■ Magnox - are pressurized, carbon dioxide cooled, graphite moderated reactor■ Advanced gas-cooled reactor (AGR)
3. Shrinkage of Material
There are three types of shrinkage: (i) Shrinkage of the liquid, (ii) Solidification shrinkage and(iii) Patternmaker's shrinkage. The shrinkage of the liquid is rarely a problem because more material is flowing into the mold behind it. Solidification shrinkage occurs because metals are less dense as a liquid than a solid, so during solidification the metal density dramatically increases. Patternmaker's shrinkage refers to the shrinkage that occurs when the material is cooled from the solidification temperature to room temperature, which occurs due to thermal contraction.An equation of state can be used to predict the values of the thermal expansion at all the required temperatures and pressures, along with many other state functions.In the major equations of state, if for a given amount of substance contained in a system, the temperature, volume, and pressure are not independent quantities; they are connected by a relationship of the general form: | (1) |
In the following equations the variables are defined as follows. Any consistent set of units may be used, although SI units are preferred. Absolute temperature refers to use of the Kelvin (K) or Rankine (°R) temperature scales, with zero being absolute zero.P = pressure (absolute)V= volumeN = number of moles of a substanceVm= V/n = molar volume, the volume of 1 mole of gas or liquidT = absolute temperatureR = ideal gas constant (8.314472 J/(mol·K))Pc= pressure at the critical pointVc= molar volume at the critical pointTc= absolute temperature at the critical pointThe classical ideal gas law may be written as: | (2) |
The ideal gas law may also be expressed as follows | (3) |
where, P is the density,
is the adiabatic index (ratio of specific heats), e = CvT is the internal energy per unit mass (the "specific internal energy"), Cv is the specific heat at constant volume, and Cp is the specific heat at constant pressure.
4. Linear Expansion
To a first approximation, the change in length measurements of an object ("linear dimension" as opposed to, e.g., volumetric dimension) due to thermal expansion is related to temperature change by a "linear expansion coefficient". It is the fractional change in length per degree of temperature change. Assuming negligible effect of pressure, we may write: | (4) |
where L is a particular length measurement and
is the rate of change of that linear dimension per unit change in temperature.The change in the linear dimension can be estimated to be: | (5) |
This equation works well as long as the linear-expansion coefficient does not change much over the change in temperature ΔT. If it does, the equation must be integrated.
5. Effects on Strain
For solid materials with a significant length, like rods or cables, an estimate of the amount of thermal expansion can be described by the material strain, given by and defined as: | (6) |
where Linitial is the length before the change of temperature and Lfinal is the length after the change of temperature.For most solids, thermal expansion is proportional to the change in temperature: | (7) |
Thus, the change in either the strain or temperature can be estimated by: | (8) |
 | (9) |
is the difference of the temperature between the two recorded strains, measured in degrees Celsius or Kelvin, and αL is the linear coefficient of thermal expansion in "per degree Celcius" or "per Kelvin", denoted by °C−1 or K−1, respectively.
6. Area Expansion
The area thermal expansion coefficient relates the change in a material's area dimensions to a change in temperature. It is the fractional change in area per degree of temperature change. Ignoring pressure, we may write: | (10) |
where A is some area of interest on the object, and
is the rate of change of that area per unit change in temperature.The change in the linear dimension can be estimated as: | (11) |
This equation works well as long as the linear expansion coefficient does not change much over the change in temperature δT. If it does, the equation must be integrated.
7. Volumetric Expansion
For a solid, we can ignore the effects of pressure on the material, and the volumetric thermal expansion coefficient can be written:  | (12) |
where V is the volume of the material, and
is the rate of change of that volume with temperature.This means that the volume of a material changes by some fixed fractional amount. For example, a steel block with a volume of 1 cubic meter might expand to 1.002 cubic meters when the temperature is raised by 50°C. This is an expansion of 0.2 %. If we had a block of steel with a volume of 2 cubic meters, then under the same conditions, it would expand to 2.004 cubic meters, again an expansion of 0.2%. The volumetric expansion coefficient would be 0.2% for 50K, or 0.004%/K.If we already know the expansion coefficient, then we can calculate the change in volume | (13) |
where
is the fractional change in volume (e.g., 0.002) and ΔT is the change in temperature (50 C).The above example assumes that the expansion coefficient did not change as the temperature changed. This is not always true, but for small changes in temperature, it is a good approximation. If the volumetric expansion coefficient does change appreciably with temperature, then the above equation will have to be integrated: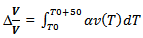 | (14) |
where To is the starting temperature and
is the volumetric expansion coefficient as a function of temperature T.The temperature of the fuel varies as a function of the distance from the center to the rim. At distance d from the center the temperature (Td) is described by the equation where
is the thermal conductivity. | (15) |
When the nuclear fuel increases in temperature, the rapid motion of the atoms in the fuel causes an effect known as Doppler broadening. When thermal motion causes a particle to move towards the observer, the emitted radiation will be shifted to a higher frequency. Likewise, when the emitter moves away, the frequency will be lowered. For non-relativistic thermal velocities, the Doppler shift in frequency will be: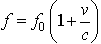 | (16) |
where f is the observed frequency, f0 is the rest frequency, υ is the velocity of the emitter towards the observer, and c is the speed of light.Since there is a distribution of speeds both toward and away from the observer in any volume element of the radiating body, the net effect will be to broaden the observed line. If Pυ(υ)dυ is the fraction of particles with velocity component υ to υ+dυ along a line of sight, then the corresponding distribution of the frequencies is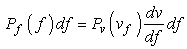 | (17) |
where
is the velocity towards the observer corresponding to the shift of the rest frequency
to
. Therefore,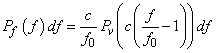 | (18) |
We can also express the broadening in terms of the wavelength
. Recalling that in the non-relativistic limit
, we obtain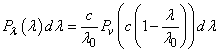 | (19) |
In the case of the thermal Doppler broadening, the velocity distribution is given by the Maxwell distribution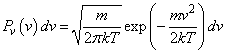 | (20) |
where,m is the mass of the emitting particle, T is the temperature and k is the Boltzmann constant.Then,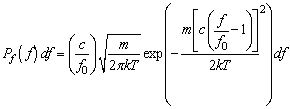 | (21) |
We can simplify this expression as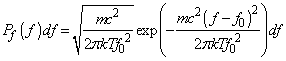 | (22) |
which we immediately recognize as a Gaussian profile with the standard deviation | (23) |
and full width at half maximum (FWHM)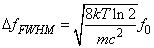 | (24) |
The fuel then sees a wider range of relative neutron speeds. Uranium-238, which forms the bulk of the uranium in the reactor, is much more likely to absorb fast or epithermal neutrons at higher temperatures. This reduces the number of neutrons available to cause fission, and reduces the power of the reactor. Doppler broadening therefore creates a negative feedback because as fuel temperature increases, reactor power decreases. All reactors have reactivity feedback mechanisms, except some gas reactor such as pebble-bed reactor which is designed so that this effect is very strong and does not depend on any kind of machinery or moving parts.
8. Accident Analysis
Several reports on the safety of graphite moderated reactors include “Resolution of Generic Safety Issues”[3], "Evaluation of graphite safety issues for the British production piles at Windscale"[4], “Properties of ATR-2E Graphite and Property Changes due to Fast Neutron Irradiation”[5], “Management of ageing in graphite reactor cores”[6] and "Meeting of RG2 with Windscale Pile 1 Decommissioning Project Team"[7].These accidents may be as a result of design concept process of some of these reactors (which could involve novel technologies) that have inherent risk of failure in operation and were not well studied/understood. In avoiding such accidents the nuclear industry has been very successful. As in over 14,500 cumulative reactor-years of commercial operation in 32 countries, there have been only three major accidents to nuclear power plants – Fukushima, Chernobyl and Three Mile Island. As in other industries, the design and operation of nuclear power plants aims to reduce the likelihood of accidents, and avoid major human consequences when they occur.However, recent study of the reactor fuel under accident conditions, reveal that after subjecting the fuel to extreme temperatures — far greater temperatures than it would experience during normal operation or postulated accident conditions — TRISO fuel is even more robust than expected. Specifically, the research revealed that at 1,800 degrees Celsius (more than 200 degrees Celsius greater than postulated accident conditions) most fission products remained inside the fuel particles, which each boast their own primary containment system[8].
9. Risk Analysis
In physics, risk can be measured in terms of frequency and magnitude. The primary causes of engineering disasters are usually considered to be: human factors (including both 'ethical' failure and accidents) design flaws (many of which are also the result of unethical practices) materials failures extreme conditions or environments, and, most commonly and importantly - combinations of these reasons. It is very clear from the Table 1 that insufficient knowledge top the table of reason for failure with 36%, while 16% was recorded for Underestimation of influence as reason for failure. Ignorance, carelessness, negligence have 14% and Forgetfulness, error scored 13%. Whatever may be the reasons for failure, no amount of risk can be ignore on equipment or system in operation, therefore, nuclear reactor safety must be well study.Table 1. Highlighted recent study conducted at the Swiss Federal Institute of Technology in Zurich on reasons for failure in which 800 accidents analysed 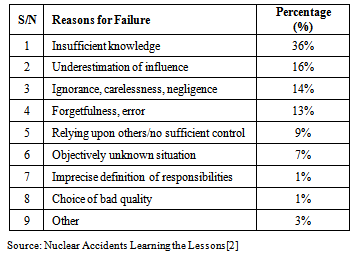 |
| |
|
Also, failure may be evaluated by measures of risks which include performance, obsolete components, wrong application, errors and accident. These risks can be defined and quantified as the product of the probability of an occurrence of failure and a measure of the consequence of that failure. Since the objective of engineering is to design and build things to meet requirements, apart from cost implication, it is important to consider risk along with performance, and technology selections made during concept design. Engineering council guidance on risk for the engineering profession defined “Engineering Risk” as “the chance of incurring a loss or gain by investing in an engineering project”. Similar definitions are given by Modarres, Molak and Blanchard that risk is a measure of the potential loss occurred due to natural or human activities.
10. Future Reactors
Graphite-moderated helium-cooled reactors such as the Pebble Bed Modular Reactor (PBMR) and prismatic-fuel high-temperature gas reactor (HTGR) may represent the future of nuclear power. As they claim to have high efficiency, intrinsic safety and ability to utilise a variety of nuclear fuel types. There have been several concept design studies relating to HTGR, including a study of the gas turbine systems that would be required to achieve the high efficiency promised by these high-temperature reactors.More likely that future reactor could potentially be fuelled with thorium, an abundant fertile nuclear fuel, providing even longer-term security of energy supply. There are also some safety benefits to the Thorium cycle. Currently there seem to be an international debate regarding the technical and commercial viability of various Thorium-fuelled reactor concepts. Thorium fuel could potentially be used in various reactor concepts, primarily Graphite-Moderated Helium - Cooled reactors, but also potentially Water-Cooled Reactors (of various types) or Fast Reactors. There has been a Molten-Salt-Liquid-Fuel Thorium Reactor concept demonstrated in the past.
11. Methodology
In this work, Ordinary Least Square (OLS) methodology, which is largely used in nuclear industry for modeling safety, is employed. Some related previous works on the application of regression analysis technique include: “Advances in high temperature nuclear reactor fuel”[9], “Stochastic Modeling of Deterioration in Nuclear Power Plants Components”[10], “Regression Approach to a Simple Physics Problem”[11], “Best estimate safety analysis for nuclear power plants uncertainty evaluation"[12]. Others are, “Estimation of the power peaking factor in a nuclear reactor using support vector machines and uncertainty analysis”[13], “Regression analysis of gross domestic product and its factors in Lithuania”[14]. An Approach for validating actinide and fission product burnup credit criticality safety analyses isotopic composition predictions”[15], “Extending the application range of a fuel performance code from normal operating to design basis accident conditions”[16] and “Recent research Investigating the Effect ofLoss-of-Pressure-Control on the Stability of Water-Cooled Reactor Design Models”[17].
12. The Research Objectives
To apply the linear regression technique on reactor design models such as a typical or experimental Graphite Moderated Reactor Design (GMRD) models for the determination of their Safety Margin in terms of applicable fuel size or fuel volume under a particular temperature within the operating reactor core and to carry out analysis of the reactor stability on the rate of fuel size or fuel application with respect to design parameter of the reactor graphite core height and diameter.
13. The Research Motivation
The purpose of this work is to assist countries wishing to include nuclear energy for the generation of electricity, like Nigeria, to secure a reactor that is better and safe. Also, the studies intended to provide guidance in developing practical catalytic materials for power generation reactor and to help researchers make appropriate recommendation for Nigeria nuclear energy proposition as one of the solutions to Nigeria energy crisis. Moreover, the study is to provide a good, novel approach and method for multi-objective decision-making based on six dissimilar objectives attributes: evolving technology, effectiveness, efficiency, cost, safety and failure. Furthermore, this is to help such country meet their international obligations to use nuclear technology for peaceful means and for economic development for mankind. Finally, the achievement is to make worldwide contribution to knowledge.
14. Research Design/Approach
Theory and experiment have shown that for a water-cooled reactor, the volume of fuel determines the heat or decay heat within reactor core. Therefore, the mass of the fuel plays significant role in the safety of the reactor during operation in preventing overheating of the reactor and reactor meltdown during accident. Hence, in this work, an assessment of the rise in fuel temperature in the reactor is considered of a typical boiling/pressurized water reactor designs. More specifically, the studies will concentrate on technical factors that limit the achievement of higher burn-up of fuel, such as the fuel size mechanical interaction. Detailed investigations of fuel behaviour under reactor accident conditions are also included. Table 2. Input data for safety margin against graphite height and graphite diameter in a typical graphite moderated reactor design model 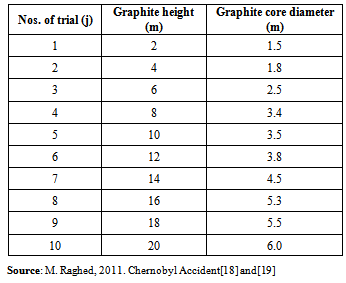 |
| |
|
The research approach involves adjusting the parameters of a model function to best fit a data set. A simple data set consists of n points (data pairs)
, i = 1, ..., n, where
is an independent variable and
is a dependent variable whose value is found by observation. The model function has the form f (x,β), where the m adjustable parameters are held in the vector β. The goal is to find the parameter values for the model which "best" fits the data. The least squares method finds its optimum when the sum, S, of squared residuals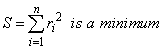 | (25) |
The Tables 2 presented the values of design fuel input parameters in an operating reactor. For each of these different designs, a linear regression analysis technique was applied using statistical power analysis software, NCSS.
15. Results and Analyses
1. Graphite Moderated Reactor Design (GMRD) The results of the application of the linear regression analysis of the data in Table 2 for a typical Graphite Moderated Reactor Design (GMRD) models are presented as follows:(i) Empirical Expression for Safety Factor, ỲExamine the effect of fuel size on the Stability and Safety of the nuclear reactor during operation. The data obtained in Table 1 which represents a typical parameter for Graphite Moderated Reactor Design (GMRD) models was modified in order to obtain the best fit for the model. The new conceptual fuel design for reactor operation could optimize the performance of the water-cooled reactor.The linear regression model equation to be solved is given by: 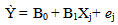 | (26) |
where, B0 is an intercept, B1 is the slope, Xj is the rate of increase in fuel volumeej = error or residual, j = 1,2,3,…,k and k is the last term.Empirical Expression for Safety Factor, Ỳ for Normal Pressure Reading The model empirical expression is the equation of the straight line relating heat in the reactor and the volume of fuel in the reactor as a measure of safety factor estimated as: | (27) |
- the equation (27) is the estimated model or predicted where, Ỳ = Dependent Variable, Intercept = 0.9583, Slope = 0.2575, X = Independent Variable, e = error or residual, j = 1,2,3,…,10 and 10 is the last term of trial.The Figure 1 shows the linear regression plot section of residual of heat or fuel temperature and volume of the fuel effect on the reactor(ii) Linear regression plot section 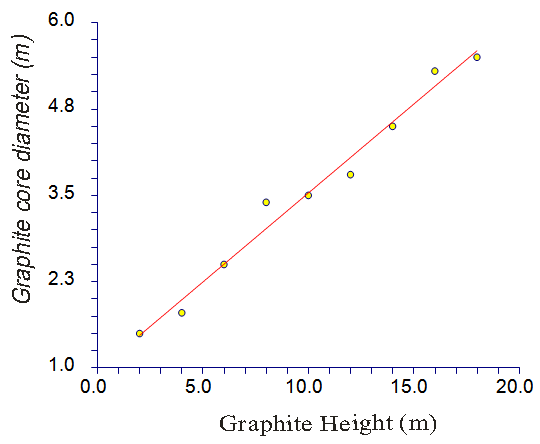 | Figure 1. Graphite height and diameter effect on the stability of operating reactor |
(iii) F-test ResultTable 3. Summary of F-test Statistical Data 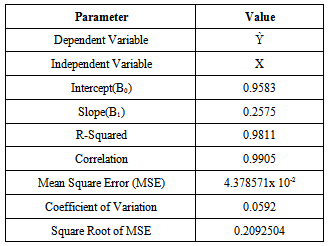 |
| |
|
In Table 3 the R2 value of 0.9811 indicates that 98.11% of the variation in Y has been explained by the X variables.Siegel (2012, P 577) has shown that R2 can be used to test the validity of a model since it can be tested directly in this manner. If R2 calculated value is smaller than the critical value in the R2 table then the model is not significance in that case we accept Ho. But, if the R2 value is larger for the calculated value, then the model is significant at the given significant level. The critical value for n-12 and k-1 is 0.673 or 67.3%. Thus the model equation is significant at the given significant level of 5%.The correlation at 0.9905 shows that the model has significant level of acceptance and could be of significant practical application. Accounting for 0.95% safety marginThe value 4.378571x 10-2 for the mean square error (MSE) indicates that the error is minimized at optimal. This value 4.378571x 10-2 shows that the error is high in the test.The Table 4 highlights descriptive statistics section results Table 4. Descriptive Statistics Section 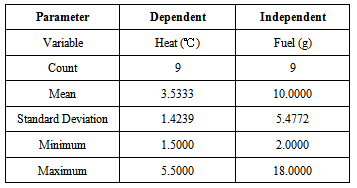 |
| |
|
The Table 5 is the regression estimation section results that show the least-squares estimates of the intercept and slope followed by the corresponding standard errors, confidence intervals, and hypothesis tests. These results are based on several assumptions that are validated before they are used. In Table 6 the analysis of variance shows that the F-Ratio testing whether the slope is zero, the degrees of freedom, and the mean square error. The mean square error, which estimates the variance of the residuals, was used extensively in the calculation of hypothesis tests and confidence intervals.Table 5. Regression Estimation Section 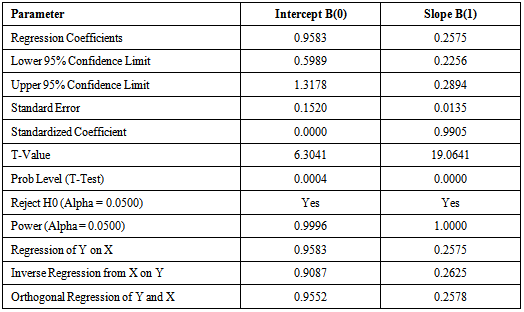 |
| |
|
Table 6. Analysis of Variance Section 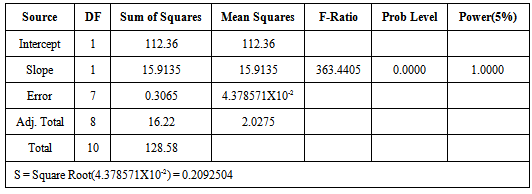 |
| |
|
In Table 7 Anderson Darling method confirms the rejection of H0 at 20% level of significance but all of the above methods agreed that H0 Should not be rejected at 5% level of significance. Hence the normality assumption is satisfied as one of the assumptions of the Linear Regression Analysis is that the variance of the error variable δ2 has to be constant.Table 7. Tests of Assumptions Section 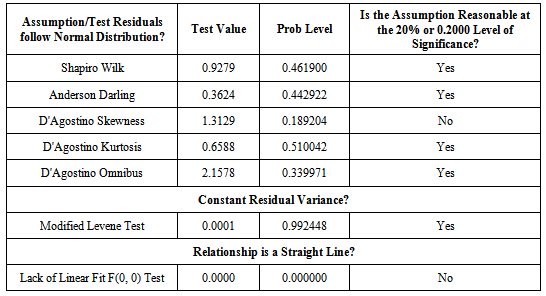 |
| |
|
Notes:A 'Yes' means there is not enough evidence to make this assumption seem unreasonable.A 'No' means that the assumption is not reasonable.(iv) Residual Plots SectionThe plot section is used as further check on the validity of the model to satisfy all the assumptions of the linear regression analysis. Amir D. Aczel (2012, P528). Entanglement: The Greatest Mystery in Physics, have stated that the normality assumption can be checked by the use of plot of errors against the predicted values of the dependent variable against each of the independent variable and against time (the order of selection of the data points) and on a probability scale.The diagnostic plot for linear regression analysis is a scatter plot of the prediction errors or residuals against predicted values and is used to decide whether there is any problem in the data at hand Andrew F. Siegel (2012, p.578). The Figure 2 is for the plot of errors against the order to selection of the data points (e = 1,2,…,12). Although the order of selection was not used as a variable in the mode, the plot reveal whether order of selection of the data points should have been included as one of the variables in our regression model. This plot shows no particular pattern in the error as the period increases or decreases and the residuals appear to be randomly distributed about their mean zero, indicating independence. The residuals are randomly distributed with no pattern and with equal variance as volume of fuel increases. Figure 2 presents Residuals of Heat (℃) against Fuel (g)Note: 1. Residual = original value for heat (Y) minors predicted value for heat, Ỳ 2. Count = the design number (design 1, 2, 3, …, 12 )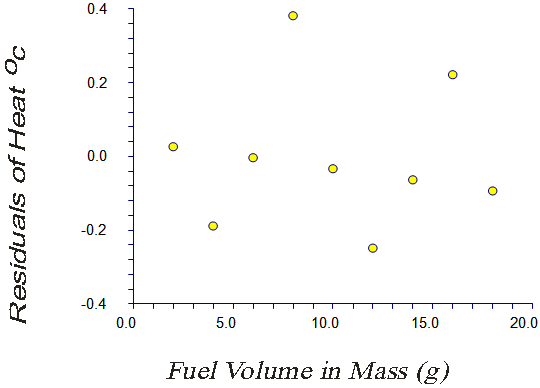 | Figure 2. Residuals of Heat (℃) versus Fuel (g) |
Figure 3 presents the histogram of residuals of error (et) and this is nearly skewed to the right but the software used indicated that the plot is normal.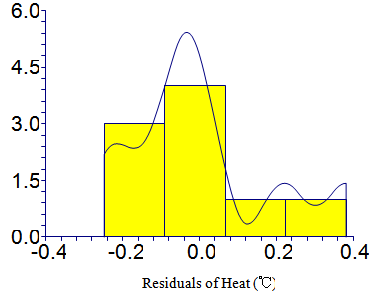 | Figure 3. Histogram of Residuals of Heat (℃) |
Figure 4 is the result on plot graph of experimental errors. The residuals are perfectly normally distributed as most of the error terms align themselves along the diagonal straight line with some error terms outside the arc above and below the diagonal line. This further indicates that the estimated model is valid. 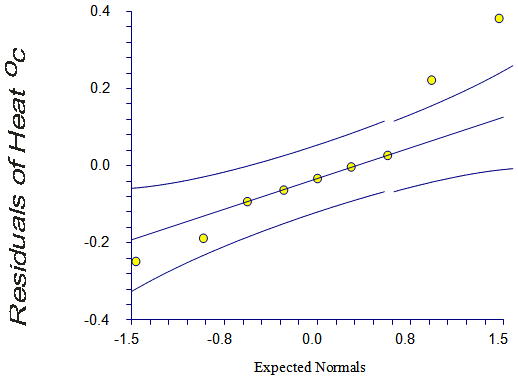 | Figure 4. Normal Probability Plot of Residuals of Heat (0C) |
16. Summary/Conclusions
In summary, the equation of the straight line relating heat in the reactor as against the volume of fuel in the reactor is estimated as:
- this is the estimated model or predicted(i) This is the model equation that could be applied to make predictions of the safety factor on these types of reactor design models as relating to the heat in the reactor and the volume of fuel in the reactor.(ii) The empirical expressions may also be used for the calculation of heat (℃), Ỳ in the reactors which in turn is a measure of the reactor’s stability.(iii) Also, the empirical formula derived can be used to determine the contribution of heat or temperature (℃) to the stability of the reactor during operation or accident. (iv) The estimated value of Ỳ when Xj is zero is 0.9583 with a standard error of 0.1520. (v) The y-intercept, the estimated value of h when r is zero, is 0.9583 with a standard error of 0.1520.(vi) The slope represent the estimated change in heat (Ỳ) per unit change in fuel (Xj), is given as 0.2575 with a standard error of 0.1520. (vii) The value of coefficient of determination (R2) explains the proportion of the variation in heat that can be accounted for by variation in fuel as 0.9811. (viii) The correlation between heat (Ỳ) and fuel (Xj) is 0.9905.(ix) A significance test that the slope is zero resulted in a t-value of 19.0641. The significance level of this t-test is 0.0000. Since 0.0000 < 0.0500, the hypothesis that the slope is zero is rejected.(x) The estimated slope is 0.2575. The lower limit of the 95% confidence interval for the slope is 0.2256 and the upper limit is 0.2894. (xi) The estimated intercept is 0.9583.(xii) The lower limit of the 95% confidence interval for the intercept is 0.5989 and the upper limit is 1.3178.In conclusion the results of the statistical analysis on these types of nuclear reactor models reveals that the GMRD models promises stability under application of large size of graphite at a height of 10.0metre and diameter of 3.5metre. At this point the temperature seems at maximum and the reactor agrees to be most stable as the regression plot was optimized, that is the least squares method finds its optimum when the sum, S, of squared residual
is a minimum at the given dimension of graphite core height 10.0metre and diameter 3.5metre. Meanwhile, at anything below height of 10.0metre and diameter of 3.5metre the fuel element seems to be unstable in the reactor as the regression plot could not find it optimal. The research implication is that the design dimension of graphite moderated reactor core could be significant to the nuclear fuel temperature and safety of the reactor during operation or accident. Secondly, the safety margin prediction of up to 0.95% has been validated for reactor design models on water-cooled reactor regarding the design dimension of graphite moderated reactor core parameter, core temperature and fuel temperature. The research effort served as an advantage over the current 5.1% challenging problem for plant engineers to predict the safety margin limit. According to Xianxun Yuan (2007, P49) in “Stochastic Modeling of Deterioration in Nuclear Power Plants Components” a challenging problem of plant engineers is to predict the end of life of a system safety margin up to 5.1% validation. The current design limits for various reactors safety in a nuclear power plant, defined by the relative increase and decrease in the parametric range at a chosen operating point from its original value, varies from station to station.However, the finding in the work would suggest that the design of the plant should ensure that operating reactor core are made up of large graphite core in order to minimize core melting in an extreme high temperature condition which can damaged the reactor. Therefore, the design of the reactor graphite diameter should be up to 3.5metre and the design of the reactor graphite core height up to 10.0metre that would withstand and secure high temperature during operation or accident for the safety of the reactor. In light water graphite reactors certain factors favour the choice of this type of reactor, firstly the main element of the core is an independent fuel channel there is no definite upper limit to capacity as additional channels could be added to the design. Secondly, the component being modular could be manufactured at existing facilities. Thirdly, refueling online is possible with independent fuel channels leading to high load factors subsequent operation indicated that design reserves is more than adequate and that with minimal changes; power could be increased in subsequent designs of the some physical size. Though one disadvantage of the light water graphite reactors may be that steam is generated in the reactor core.Nevertheless, the discoveries on high temperature effect on graphite moderated reactor operational stability and safety, either in terms of design specification of reactor graphite core dimension parameter or on fuel temperature effect should provide a new method for reactor design concept. This shall also provide a good, novel approach and method for multi-objective decision-making based on six dissimilar objectives attributes: evolving technology, effectiveness, efficiency, cost, safety and failure. The implication of this research effort to Nigeria’s nuclear power project drive.It is therefore recommended that for countries wishing to include nuclear energy for the generation of electricity, like Nigeria, the design input parameters of the selected nuclear reactor should undergo test and analysis using this method for optimization and choice.
ACKNOWLEDGMENTS
We thank Department of Physics, Nigerian Defence Academy (NDA) Kaduna, Nigeria Atomic Energy Commission (NAEC), Energy Commission of Nigeria (ECN), National Open University of Nigeria (NOUN), Department of Science and Information Technology and Department of Mathematics, Ahmadu Bello University (ABU) Zaria, Nigeria, for the human and material support during the research work.
References
[1] | Marlin P. Metcalfe and John F.B. Payne 2010, The applicability of the equivalent temperature concept for the correlation of graphite dimensional change behavior in magnox reactor cores. National Nuclear Laboratory, Building 102(B), Stonehouse park, Bristol Road, Stonehouse, Gloucestershire, Uk GL 10 3UT. |
[2] | Yanko Yanev. (2013), Nuclear Accidents Learning the Lessons. Atoms for Peace, NEM School Tokai Mura, Japan 2013. |
[3] | IAEA 2005, Accident analysis for nuclear power plants with graphite moderated boiling water RBMK reactors. |
[4] | U.S.NRC 2012, Resolution of Generic Safety Issues: Task CH6: Graphite-Moderated Reactors ( NUREG-0933, Main Report with Supplements 1–34). Page Last Reviewed/ Updated Thursday, March 29, 2012. |
[5] | Marsden, B.J.; Preston, S.D.; Wickham, A.J., 2010, Evaluation of graphite safety issues for the British production piles at Windscale, AEA Technology (IAEA). IAEA-TECDOC—1043. Retrieved 13 November 2010. |
[6] | Haag, G.2005 “Properties of ATR-2E Graphite and Property Changes due to Fast Neutron Irradiation,” FZ-Juelich, Juel-4813, 2005. |
[7] | Gareth B. Neighbour. (2007), Management of ageing in graphite reactor cores,” Royal Society of Chemistry, 2007. ISBN 978-0-85404-345-3. Retrieved 2009-06-15. |
[8] | "Meeting of RG2 with Windscale Pile 1 Decommissioning Project Team," Nuclear Safety Advisory Committee. 2005-09-29. NuSAC(2005) P 18. Retrieved 2008-11-26. |
[9] | Rod Adams, “Advances in high temperature nuclear reactor fuel – TRISO integrity at 1800 C,” The Idaho National Laboratory released the following exciting news on September 25, 2013. |
[10] | X. Yuan, “Stochastic Modeling of Deterioration in Nuclear Power Plants Components” Ph. D. University of Waterloo, Ontario, Canada. |
[11] | J. Peltier, “Regression Approach to a Simple Physics Problem,” Peltier Technical Services, Inc., Copyright © 2011. |
[12] | IAEA, “Safety reports series no.52 best estimate safety analysis for nuclear power plants uncertainty evaluation,” International Atomic Energy Agency, VIENNA, 2008. |
[13] | Inhobae, “Estimation of the power peaking factor in a nuclear reactor using support vector machines and uncertainty analysis,” Department of Nuclear Engineering, Chosun University 375 Seosuk-dong, Dong-gu, Gwangju 501-759, Korea, 2008. |
[14] | Viktorija Bobinaite, “Regression analysis of gross domestic product and its factors in Lithuania,” Kaunas University of Technology, Lithuania Published by ISSN 1822-6515 economics and management: 2011. |
[15] | G. Radulescu, “An Approach for validating actinide and fission product burnup credit criticality safety analyses isotopic composition predictions,” NUREG/CR 7108 ORNL/ TM 2011/509. Manuscript Completed: Published: April 2012. |
[16] | Van Uffelen, P., “Extending the application range of a fuel performance code from normal operating to design basis accident conditions,” Journal of Nuclear Materials, 2008. 383: pp. 137-143. |
[17] | Oludare A.I., Agu N.M., Akusu P.O., Omolara E.O., Umar A.M. Akusu C.O., Investigating the Effect of Loss-of-Pressure-Control on the Stability of Water-Cooled Reactor Design Models,” International Journal of Energy and Environmental Research Vol.1, No.1, pp.1-15, September 2013. |
[18] | M. Raghed. (2011), Chernobyl Accident” September 2011. |
[19] | R.A. Chapin. (2011), Nuclear Energy Materials & Reactors Vol.II. Light Water Grapghite Reactors. Department of Chemical Engineering, University of New Brunshwick, Canada, 2003. |
[20] | Amir D. Aczel. (2011), Entanglement: The Greatest Mystery in Physics. Published on October 1, 2012. |
[21] | Andrew F. Siegel, “Practical Business Statistics,” Publication: New Delhi Elsevier 2012. |