Beckley Ikhajiagbe 1, Geoffrey O. Anoliefo 1, Osazuwa Omoregbee 2, Paula Osigbemhe 2
1Department of Plant Biology and Biotechnology, University of Benin, Nigeria
2Department of Science Laboratory Technology, University of Benin, Nigeria
Correspondence to: Beckley Ikhajiagbe , Department of Plant Biology and Biotechnology, University of Benin, Nigeria.
Email: |  |
Copyright © 2012 Scientific & Academic Publishing. All Rights Reserved.
Abstract
This study was conducted to investigate the effect of heat shock in the remediation of poly aromatic hydrocarbons (PAHs) and heavy metals of a waste engine oil-polluted soil. This was also subjected to phytoassessment using Vigna unguiculata cv Kano white. Top soil (10kg) was collected and thoroughly mixed with waste engine oil (WEO) on weight basis, to obtain 5% w/w oil-in-soil. The buckets were divided into six sets, each receiving heat treatment at different times; twice per week (2PW), once a week (1PW), once in a fortnight (1PF) and once in a month (1PM). There were 2 controls which did not receive any heat treatment; the unpolluted soil (CTRL) and the polluted soil (NON). Heat was applied by carefully burying a stainless steel container, which received boiling water (100℃) at the designated intervals. The set up was allowed to remain in a well ventilated screen house with inherent room temperature for 3 months. Results showed significant reductions in heavy metal contents after the heat treatment. Heavy metal remediations were better in 1PM and 1PF. Total PAH was the lowest in 1PM (154.51mg/kg), compared to 504.48mg/kg in NON. Comparatively, the most frequent the heat shock received by soil, the lower the rate of remediation. Results of phytoassessment using cowpea also showed improved growth and yield parameters in the less frequently heated oil-polluted buckets, compared to the unheated ones (NON).
Keywords:
Bioremediation, Cowpea, Hazard quotient, Heat shock, Natural attenuation, PAH, Petroleum hydrocarbon, Phytoassessment
Cite this paper: Beckley Ikhajiagbe , Geoffrey O. Anoliefo , Osazuwa Omoregbee , Paula Osigbemhe , Changes in the Intrinsic Qualities of a Naturally Attenuated Waste Engine Oil Polluted Soil after Exposure to Different Periods of Heat Shock, Resources and Environment, Vol. 4 No. 1, 2014, pp. 45-53. doi: 10.5923/j.re.20140401.05.
1. Introduction
Oil exploration and exploitation in many countries have brought about environmental pollution and serious public health concern, which has established the need to create a wide variety of innovative chemical, physical and biological processes that can get rid of hazardous organics from the environment without causing further ecological damage. Remediating a site polluted with hazardous waste materials is a very tedious and complex procedure; and this usually involves a systematic, step-by-step problem solving approach. Bioremediation of contaminated soils is currently regarded as one of the most successful ways to clean up contaminated sites, particularly because it is adjudged ecofriendly. According to bioremediation techniques[1], biological materials and processes are used to reed the soil of contaminants or to reduce the concentration of hazardous pollutants at contaminated sites. Economically, some bioremediation procedures are quite expensive, considering the cost of acquisition of necessary materials as well as remediation design. There exists, however, a very cost effective and very reliable means of bioremediation that utilizes the soil’s inherent intrinsic capabilities for remediating contaminants without having to be necessarily modified by human activity. The process is called natural attenuation.Soil microorganisms play a very important role in the intrinsic bioremediation of contaminants. Themicroorganisms break down contaminants by using them as a food source or cometabolizing them with a food source. Microorganisms can be isolated from almost any environmental conditions. Microbes will adapt and grow at subzero temperatures, as well as extreme heat, desert conditions, in water, with an excess of oxygen, and in anaerobic conditions, with the presence of hazardous compounds or on any waste stream. Field temperatures play a significant role in controlling the nature and extent of hydrocarbon metabolism. Temperature affects the rate of biodegradation, as well as the physical nature and chemical composition of hydrocarbons[1]. The main requirements are an energy source and a carbon source. Because of the adaptability of microbes and other biological systems, these can be used to degrade or remediate environmental hazards.One of the important factors controlling activity and survival of microorganisms is soil temperature. Temperature affects the rate of organic matter decomposition among all the ecological factors. Temperature affects biochemical reactions rates, and the rates of many of them double for each 10℃ rise in temperature. It also determines the rate of biological degradation processes in the soil, as well as the soil moisture content[2]. Temperature increases have been found to increase the rate of degradation of organic compounds in soil[3].Thibault and Elliott[4] reported that the growth of micro-organisms usually doubles for every 10℃ rise in temperature. With increasing temperatures, adsorption decreases; and this have been reported to enhance availability of organic material for microorganisms to use for the degradation[2]. Above a certain temperature, however, the cells die. Although most microorganisms easily adapt to certain temperatures, and hence their bioremediative capabilities in these temperature-dependent situations are therefore unaffected, the present study hopes to investigate the effects of sudden temperature changes in the remediation of these oil-polluted soils. Temperature significantly affects biodegradation of hydrocarbons. Within specific range of temperature, optimum growth and activity of some microorganisms become restricted. Temperature in the thermophilic range (50 to 60℃) was shown to greatly increase decomposition of organic matter, in general. Donald[5] earlier reported that the activities of fungal and bacterial populations are inhibited by high temperatures.Given the fact that natural attenuation of contaminated soils requires a myriad of biological processes in synergism, for example, plant-direct remediation, microbial remediation, biovolatilization, bioleaching, direct or indirect impact by soil micro and macro fauna, etc, it therefore implies that whatever environmental factor, like temperature, that affects any of these processes would definitely affect the efficiency of remediation. The objectives of the present study, therefore, investigate the effects of the frequency of exposure of oil-polluted soil to temperature changes on its intrinsic remediative capabilities.
2. Materials and Methods
2.1. Sample Preparation
Top soil (0-10cm), of known chemical properties (Table 1), was collected randomly from a marked area measuring 10 x 10m on a fallow land situated near the Department of Plant Biology and Biotechnology Botanic Garden, University of Benin, Benin City, Nigeria. Thereafter, 10kg sun-dried soil was each measured into large perforated buckets with 8 random perforations made with 2 mm diameter nails at the bottom of each bowl. The buckets were 53.4 cm in height and 21.3 cm in diameter. Soil was thoroughly mixed with waste engine oil (WEO) on weight basis, to obtain 5% w/w oil-in-soil. The buckets were divided into six sets, each receiving heat treatment at different times; twice per week (2PW), once a week (1PW), once in a fortnight (1PF) and once in a month (1PM). There were 2 controls which did not receive any heat treatment; the unpolluted soil (CTRL) and the polluted soil (NON).
2.2. Methodology Adopted
Heat was applied by carefully burying a stainless steel container of boiling water (100℃), designed to fit the bucket, completely below soil surface (Fig. 1). The container, which was constructed for the purpose of the research, was 1.682 mm thick, 10 cm in diameter and 28 cm in height. The container covered half of the surface area of the soil in order to provide heat to nearly all parts of the surrounding soil. Care was taken to ensure that the water level in the container was same as that of the soil (Fig. 1). Boiling water (100℃) was poured into the container, covered with wooden lid, and then allowed to remain until after 3 hours when the water was cool. Removal was by a syringe. The container remained in position throughout the duration of the research. The set up was allowed to remain in a well ventilated screen house with inherent room temperature for 3 months. Having previously determined the soil’s water holding capacity to be 203 ml/kg soil, moisture requirements of the soils were met by wetting the soil twice a week, equally distributed, with 750 ml of water[6]. At the end of the experiment, the soil was analyzed for heavy metal and polyaromatic hydrocarbon contents.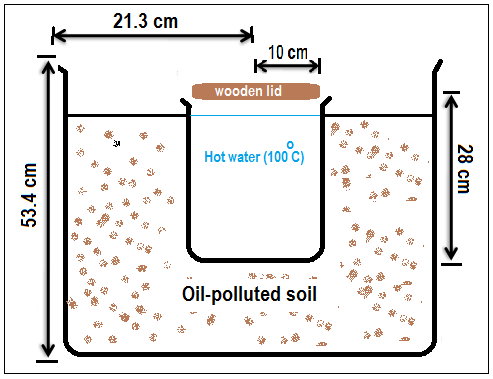 | Figure 1. A cross section of modified bucket stainless steel contained buried in oil-polluted soil |
2.3. Extraction of Micronutrients in Soils by Hydrochloric Acid Method
Ten (10) g of soil was weighed into a 250 ml plastic bottle. 100 ml of 0.1 m HCI was added, stopper, and then shaken for 30 minutes. The mixture was filtered through Whatman filter paper No.42, and then Fe, Cu, Mn, Zn, Cd, Cr, Pb, Ni, and V were determined in the filtrate by Atomic Absorption Spectrometry.
2.4. Determination of Polyaromatic Hydrocarbon Contents of Polluted Soil by Gas Chromatography (GC)
A 10 g sample was extracted with methylene chloride (DCM). The extract was filtered through anhydrous sodium sulphate to remove any trapped water molecule. This was followed by a clean- up/fractionation of the sample extract into Aliphatic and Aromatic (PAH) components. Finally, the components were concentrated using a rotary evaporator for GC analysis, using FID as detector. Model of GC used was AGILENT 6890.The GC analysis began by first injecting 1 μL of the sample extract into the GC, and the results calculated as follows:
Where, Rf = Response factor = Total Area / Total Concentration, obtained from instrument calibration with standards.Area is obtained from the chromatogram output.F.vol is the final volume of the concentrated extract (in ml)Wt is the initial weight of the homogenized sample (in grams)
2.5. Identification of Soil Microorganisms
The soil samples were air-dried and sieved through a 2 mm mesh to remove undesirable material. The dilution series for the soil sample was done by transferring 1 gram of the soil to nine (9 ml) millimetres of sterile distilled water in sterile glass containers as blank. The glass containers were shaken for 5 minutes and was taken as l0-1 dilution factor, 10 ml were then transferred from the 10-1 dilution into another 9 ml blank to obtain a 10-2 dilution and same process of transfer was repeated twice to obtain a dilution factor of 10-4.
2.5.1. Heterotrophic Bacterial and Fungal Counts
The spread plate method was employed in taking the heterotrophic bacteria counts. One (1) ml of the serially diluted portion of 10-4 of each soil sample was inoculated onto nutrient agar plates for bacteria and Potato dextrose agar plates for fungal counts. The plates were inoculated at room temperature for 24 hours and 72 hours respectively, for bacteria and fungi growth. After incubation colonies were then counted and the colony forming unit (cfu/g) of the soil samples determined.
2.5.2. Isolation of Bacterial and Fungal Oil Degraders
Bushnell- Haas (BH) medium (MgSO4, 0.20 g/I; CaCl2, 0.02 g/l; K2H,P04, 1 g/l; NH4NO3, 1 g/l; FeCl3, 0.05 g/l; KH2PO4, 1 g/l; pH 7.0, was used as the enrichment medium with 8 % (v/v) filter sterilized oil as the sole carbon source. The medium was dispensed into in 100 ml Erlenmeyer flasks and autoc1aved at 121℃ for 15 minutes. Thereafter, 5 g of each soil sample was inoculated into each flask of the medium and incubated at 130 rpm at room temperature in a HY-4 multifunctional shaker (B. Bran Scientific and Instrument Company, England). After 10 days, 1 ml of enriched media was transferred into freshly prepared enrichment media and incubated under the same conditions as described above. Serial dilutions from the third enrichment process were inoculated onto nutrient agar plates and potato dextrose agar plates for oil-degrading bacterial and funga1 counts respectively by methods described by Cowan and Steel[7] and Cheesebrough[8].
2.6. Computation of Selected Ecotoxicological Statistical Parameters
Selected parameters used in the study were Hazard quotients (HQ) as well as toxicity equivalency concentrations[6]; the object being to establish at some point whether concentrations of the contaminants after the remediative experiments could still pose any ecological threat. These were calculated as follows;
2.6.1. Hazard Quotient (HQ)
When HQ > 1: Harmful effects are likely due to contaminant in questionWhen HQ = 1: Contaminant alone is not likely to cause ecological riskWhen HQ < 1: Harmful effects are not likelyScreening benchmarks are available at Efroymson et al.[9].
2.5.2. Toxic Equivalency (TEQ) for Polycyclic Aromatic Hydrocarbons (PAH).
TEQ = ƩTi x TEF Where TEQ =Toxic Equivalency
Ti = PAH concentration in soil
TEF = Toxic Equivalency factor[10]
2.6. Phytoassessment
The success of remediation at 3 months was assessed by sowing cowpea (Vigna unguiculata cv. Kano white) in remediated soils. The plants were observed for the following parameters.
2.6.1. Assessment of Seedling Development
Percentage emergence was calculated as the percentage of seeds that sprouted above soil level of the 5 seeds originally sown per bucket. Heights were also taken by aid of a transparent calibrated ruler at 9 days after sowing (DAS). Dry weights of seedlings were taken at 9 DAS after drying seedlings in an oven at 30℃ for 3 days. Percentage survival was also calculated as the percentage of successfully emerged seedlings compared to total number of seeds sown per bowl.
2.6.2. Other Measurable Growth and Yield Parameters
Among other measurable growth parameters that were assessed during the study were shoot height, number of primary branches per plant, stem width, leaflet area, root length, number of root nodules per plant (> 3mm in diameters), number of flowers per plant, number of pods harvested per plant, number of seeds per pod, as well as100 seed weight. Grain yield per plant (g/plant) was calculated thus,
Analysis of variance in completely randomized design was done using the SPSS-15 statistical software, and means were separated by using the Least Significant Difference.
3. Results and Discussion
This study was undertaken with notion to set process conditions to reduce time required for treatment, hence reduce maintenance cost and promote smooth running of the remediation process year round especially under temperature conditions. The chemical composition of waste engine oil and top soil used for the experiment are shown in Table 1. Table 2 shows soil physiochemical parameters at three months after pollution. The total organic carbon was 2.47% in 2PW at pH of 6.85. In NON, organic carbon was 1.63% and total N was 0.13% at pH 6.99. In 1PM, total N was 0.21% at pH 6.59 while in CTRL, it was 0.14% at pH 6.03. In 1PM, Cl was 98.54 mg/kg while it was 83.04mg/kg in CTRL (Table 2).Table 1. Chemical composition of waste engine oil and top soil used for the experiment 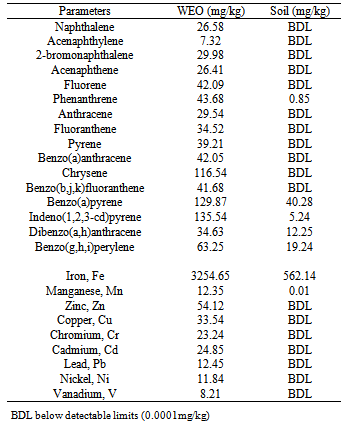 |
| |
|
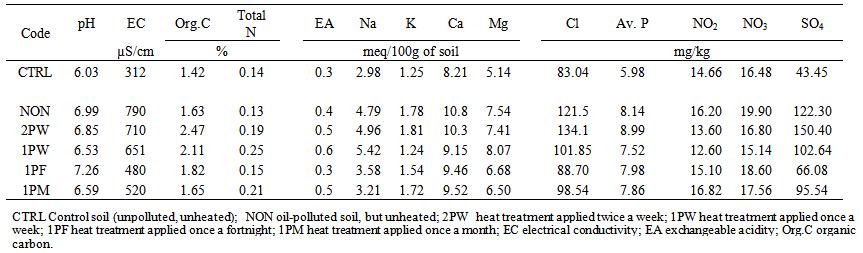 | Table 2. Soil physiochemical parameters at 3 months after soil was polluted with waste engine oil coupled with periodic exposure to heat treatments |
Values obtained for Cu in soil ranged from 30.34 -50.21mg/kg in the heat-applied treatments, compared to 54.20mg/kg in NON and 0mg/kg in CTRL (Table 3). In NON, Fe was1694.6 mg/kg, compared to V which was 4.34, making Fe the highest in concentration. In unpolluted treatment (CTRL), Cu, Cr, Cd, Pb, Ni and V were undetected. Table 4 shows hazard quotient to determine ecological toxicity of heavy metal components of waste engine oil-polluted soil at 3 months after pollution. HQ for Fe ranged from 1.48-8.47 for the five treatments plus the control, indicating that that Fe is likely to cause any harmful effects. Unlike Fe, the HQ values of Mn, Zn, Pb, and Ni were less than unity and thus these elements are not likely to cause any harmful effects. HQ of Cr in the 5 treatments ranged from 9.08-20.10, thus making Cr the metal that is most likely to cause any harmful effect (Table 4).Metals in soil have been known to be remediated by a number of mechanisms including immobilization, leaching as well as by microbial action. Metals in soil have been known to be remediated by a number of mechanisms including immobilization, leaching as well as by microbial action. The role microorganisms play in the fate of toxic metals and metalloids in the environment cannot be overemphasized; and therefore, temperature factors that hinder or enhance microbial diversity and activity will surely play a very important role in remediation. The toxicity of heavy metals, unlike organic pollutants, is intrinsic to their atomic structure and as such they cannot be transmuted or mineralized to a total innocuous form. Pollution by metals can still influence directly the biotic communities by the alteration of the abiotic conditions such as temperature and humidity. If the pollution decreases the density of the vegetation, for example, the temperature of the environment will increase and this will facilitate the increase in the diversity of thermophilic microorganisms. The results of the present study indicate that biostimulation with high temperature built up indigenous microbes in the treatments especially in 1PM treatment. The result also indicated a positive relationship between optimum temperatures and better bioremediation performance. Temperature is a strong environmental variable responsible for growth and activity of the microbes to mineralize the organic component in contaminated soil. Temperature of both air and soil has effect on the rate at which the biological degradation processes takes place in the soil, as well as the soil moisture[2,4]. Climatic factors such as moisture and temperature are often confounded in field observations of microbial activity[11, 12]. Severe drying may be lethal to bacteria, leading to the release of cell-bound P to soil and sediment[13]. The influence of temperature on microbial respiration varies with soil and environment conditions. Specifying these effects is important not only for understanding microbial dynamics under the seasonal wet–dry conditions but also for comparing data of carbon flux between different ecosystems and regions. 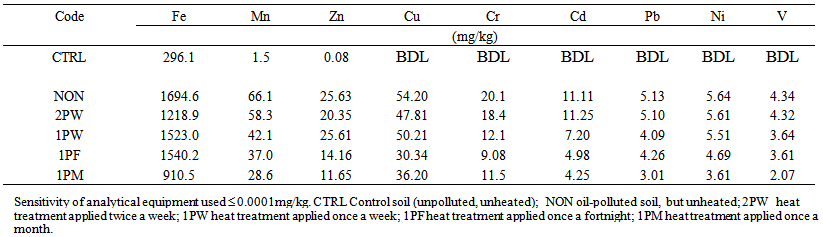 | Table 3. Heavy metal contents of soil at 3 months after pollution with waste engine oil with subsequent periodic exposure to heat treatments |
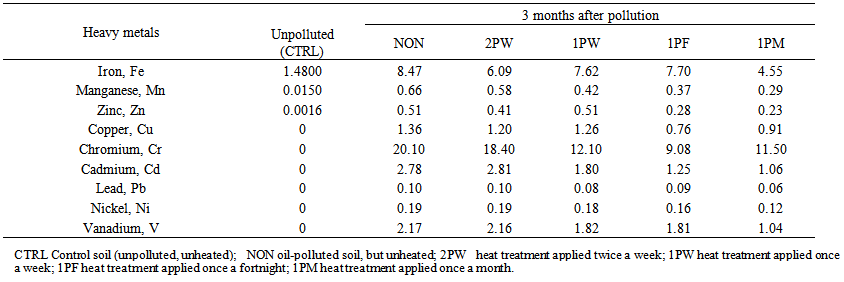 | Table 4. Hazard quotient to determine ecological toxicity of heavy metal components of waste engine oil-polluted soil at 3 months after pollution and regular exposure to heat treatments |
The hazard quotient to determine toxicity heavy metal components of WEO-polluted soil against soil microbes and microbial processes at 3 MAP have been presented on Table 5. Fe was 8.47 in NON and 4.55 in 1PM; while Cr was 2.01 in NON and 1.15 in 1PM. Fe and Cr are likely to cause harmful effects because for these two metals, HQ>1. But HQ <1 for Mn, Zn, Cu, Cd, Pb, Ni and V, and thus werelikely to cause harmful effect on microbial activity and processes.Total PAH contents of the oil-polluted soil that was never heat-treated (NON) was 504.48mg/kg (Table 6). Total PAH was lowest in 1PM (154.51mg/kg). Comparatively, the most frequent the heat shock received by soil, the lower the rate of remediation; hence efficiency of remediation followed the order 1PM >1PF>1PW>2PW>NON. Reduced frequency of heat shock improved the remediation of PAH , compared to the ( NON). This study demonstrated that temperature optimization has a positive effect on bioremediation of PAHs in contaminated soil. Lower frequencies of heat applications (1PM) showed considerable reduction in contaminants mass. At 3MAP, there was considerable increase in PAH contents of the soil which was noticed mostly in naphthalene, 2-bromonaphthalene, acenaphthene, fluorine and anthracene in the treatments 2PW, 1PW and 1PF.This increment was probably due to frequent heat shock in the soil. Also, pyrene increased from14.53mg/kg in NON to a range of 15.42 - 18.47mg/kg in the heat treatments. The best results were obtained in 1PM treatment because all the PAH components were either remediated completely or were reduced considerably. This showed that lower frequencies of heat shock were better in remediation of PAHs than when heat shock was frequently applied.Table 7 shows the hazard quotient to determine ecological toxicity of PAH components of waste engine oil-polluted soil at 3 months after pollution (MAP). HQ values for acenaphthene and fluorene were below unity (HQ<1) in all the treatments and as such could not have been said to possibly pose an ecological threat. Although HQ values were significantly high, and at toxic levels of ecological concern, there was however reduction in these values with reduced frequency of heat application, compared to the control (NON).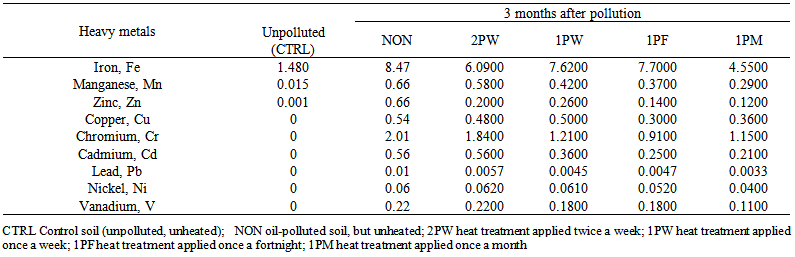 | Table 5. Hazard quotient to determine toxicity heavy metal components of waste engine oil-polluted soil against soil microbes and microbial processes at 3 months after pollution and exposure to periodic heat treatments |
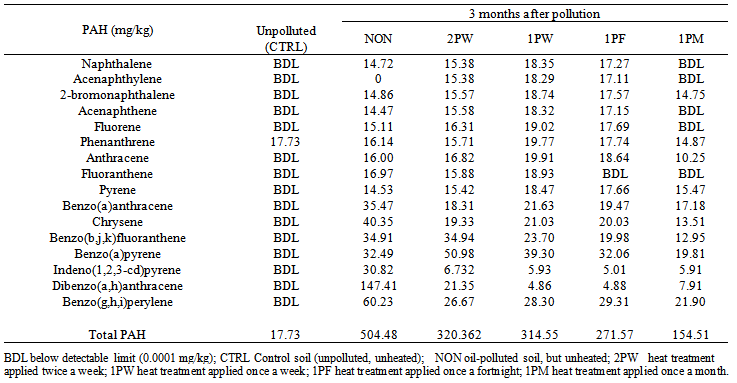 | Table 6. Polyaromatic hydrocarbon contents of oil-polluted soil exposed to periodic heat treatments for 3 months |
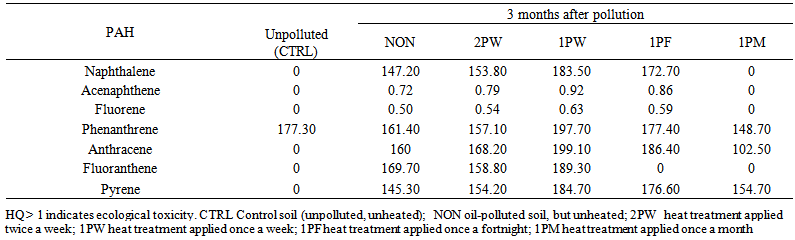 | Table 7. Hazard quotient to determine ecological toxicity of PAH components of waste engine oil-polluted soil at 3 months after pollution and periodic application of heat treatments |
Table 8 shows the concentration of toxic equivalency (TEQ) of PAH components of waste engine oil-polluted soil at 3 months after pollution. Benzo (a) pyrene was 0 g/kg in the unpolluted soil, but ranged from 19.81-50.98mg/kg in the heat-treated soils. Indeno(1, 2, 3-cd) pyrene was 3.08mg/kg in NON, it reduced to 0.50mg/kg in 1PF. total TEC reduced to 29.89mg/kg in 25% NRS-amended soils. Total TEC values in the oil-polluted soil exceeded the method B clean up level for benzo(a)pyrene (0.137mg/kg), except in the unpolluted soil (Cal-EPA, 1996).When an organic chemical is heated, its density is reduced, its vapor pressure is increased, its adsorption onto solid phases or absorption into soil organic matter is decreased, and its molecular diffusion in the aqueous and gaseous phase is also increased. The viscosity of a liquid will decrease as the temperature is increased. All these are factored into the degradability of such chemical. The diffusion coefficient in liquids is proportional to temperature. Increasing the temperature from 10°C to 100°C will increase the diffusion of a solute in the aqueous phase by approximately 30 percent. Essentially the recovery of contaminants from the subsurface can be aided by all of these changes with temperature. The thermal expansion of a liquid with its accompanying decrease in viscosity also will allow the heated liquid to flow more readily. The increased diffusion of contaminants as the temperature increases in both the aqueous and gaseous phases will help to move contaminants from areas of low permeability to areas of high permeability and speed their recovery. This forms the basis for volatility. As volatilization is the first line of elimination for low molecular weight PAHs, most lower-molecular-weight PAHs like naphthalene, acenaphthylene and fluorene are easily degraded in general[14]. Although volatilization can be influenced by soil temperature conditions as well as molecular weight of the contaminant, volatilization is also a mechanism of microbial-assisted metal. It has been reported to also result from methylation. Gharieb et al.[12] however reported that immobilization can result from sorption to cell components or exopolymers, transport into cells and intracellular sequestration or precipitation as insoluble organic and inorganic compounds, e.g. oxalates, sulphides or phosphates.Total bacterial count in oil-polluted soils ranged from 3.5 - 4.6 x 105 cfu/g, the highest value been recorded in 1PM and the least in 2PW (Table 9). Total heterotrophic bacterial count in the unpolluted soil was 5.1 x 105 cfu/g. Bacterial isolates Included Bacillus. subtilis, B. pumilis, Micrococcus luteus, Achromobacter sp, Clostridium sp, Nocardia sp, Pseudomonas sp and Sarcina sp. The highest fungal count in the heat-treated soils was obtained in 1PM (3.2 x 105 cfu/g). Microorganisms have been reported[16] to exist in many extreme environments including places where no photosynthesis occurs or where hot volcano lava is present. In these different environments, microorganisms are able to adapt and evolve over time so that they are able to live in extreme temperatures. Warmer temperatures cause many microorganisms grow faster and move more quickly. Colder temperatures do the opposite, causing the bacteria to be in a slow-moving state[16]. Microorganisms that grow optimally above 40°C are designated as thermophiles. Most thermophiles known are moderate and show an upper temperature border of growth between 50 and 70°C. Optimal growth of extreme thermophiles and hyperthermophiles occurs at 70–80°C and above 80°C, respectively[17]. Thermophiles, predominantly bacilli, possess a substantial potential for the conversion of environmental pollutants, including all major classes[18]. This explains why the PAHs and heavy metals were still remediated by these microorganisms.Percentage emergence of African yam bean seedlings at the first week after sowing ranged from 58.6 – 65.6% in the treated and untreated oil-polluted soils, compared to 98.6% in the unpolluted soil (Table 10). Although no leaf necrotic spots were recorded in the unpolluted soil, appearances of these spots were significantly delayed heat-treated soils than in the untreated oil-polluted soil. Plant growth in the oil-polluted soil was further enhanced when seeds were sown in the heat-treated soils, with better plant performances in the soils that received lower frequencies of heat shock (Table 10). Grain yield per plant, expressed as a factor of number of seeds per pod, number of pods per plant and seed dry weight was 18.00g in 1PM, 15.51g in1PF and 9.72g in the unheated oil-polluted bucket. Yield in the unpolluted soil was 27.75g/plant. This indicated that, although oil in soil hampered plant yield, those plants in exposing polluted soils to decreased frequencies of heat shocks significantly (p<0.05) enhanced crop yield.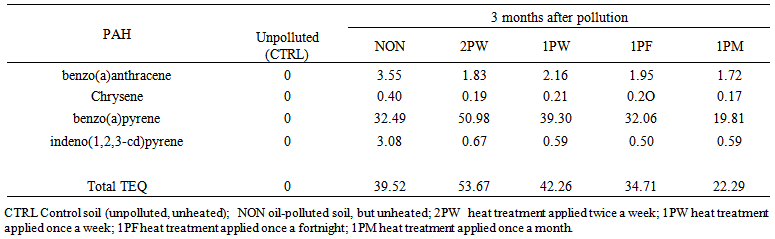 | Table 8. Toxic equivalency (TEQ) concentrations of PAH components of waste engine oil-polluted soil at 3 months after pollution and periodic exposure to heat treatments |
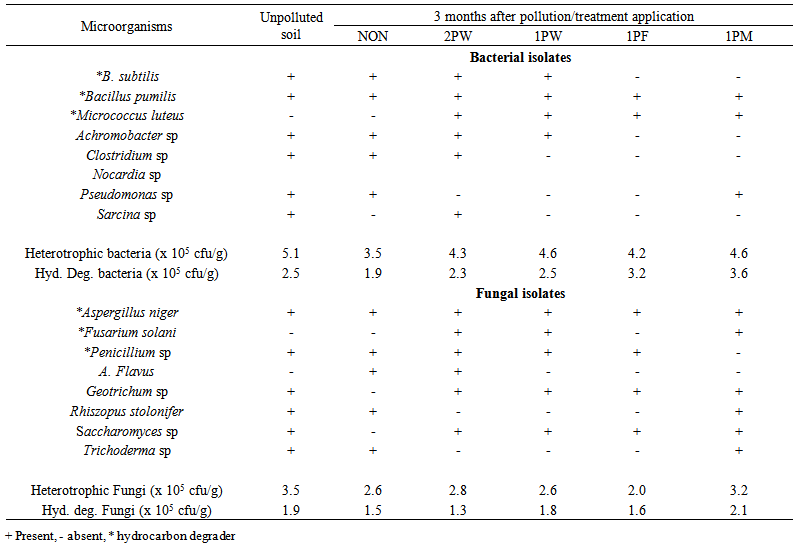 | Table 9. Microbial composition of treated and control soils after waste engine oil pollution and periodic application of heat treatments |
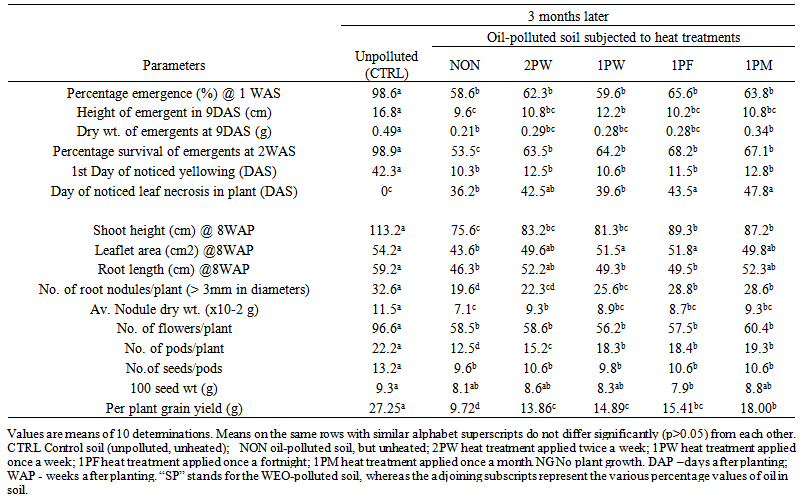 | Table 10. Some growth and yield parameters of Vigna unguiculata cv Kano white sown in heat-treated oil-polluted soils at 3 months after pollution/heat treatments |
4. Conclusions
It was obvious from the results of the laboratory experiment that temperature is one of the important variables for determining the success of bioremediation of hazardous waste. The results clearly indicated that efficiency of the bioremediation activity was enhanced with high temperature. However, the frequency of exposure of soil to such temperature has been demonstrated in this study as a factor to be reckoned with. Monthly exposure to temperatures as used in the present study has been reported as most effective. The most frequently the heat shock an oil-polluted soil receives, the less effective the rate of remediation. Phytoassessment of the remediated soils also showed comparatively improved growth and yield performances of cowpea in the less frequently heated oil-polluted buckets, compared to the unheated ones. This demonstrates that optimized temperature as well as reduced frequency of exposure speeds up the bioremediation activity in remediation systems.
References
[1] | Atlas RM, Bartha R (1992). Hydrocarbon biodegradation and soil spill. Bioremediation. In: Marshall, K (ed). Advances in Microbial Ecology Vol. 12. Plerum, New York. pp. 287-338. |
[2] | JRB Associates, Inc. 1984. Summary report: Remedial response at hazardous waste sites. prepared for municipal environment research laboratory. |
[3] | JRB Associates, Inc, 1982, Handbook of remedial action at waste disposal sites. EPA Report No. 625/6-82-006). |
[4] | Thibault GT, Elliott NW (1979). Accelerating the biological clean up of hazardous materials spills. In Proc. Oil and Haz. Mater. Spills: Prevention-Control-Cleanup-Recovery-Disposal. |
[5] | Donald LW (1994). Remediation engineering of hazardous waste contaminated soil. Marcel Dekker Inc., NY. |
[6] | Ikhajiagbe B, Anoliefo GO, Oshomoh EO and Blessing Agbonrienrien (2013). Effects of watering regimes on the intrinsic qualities of bioremediated waste engine oil-polluted soil. Annual Review and Research in Biology 3(2): 107 – 123. |
[7] | Cowan ST, Steele KJ (1974). Manual for Identification of Medical Bacteria. 2nd. Ed., Cambridge University Press, Cambridge, UK. 216p. |
[8] | Cheesebrough M (I 998). District laboratory practice in tropical countries, part II (Microbiology). Cambridgeshire Tropical Health Technology, Cambridge, UK. |
[9] | Efroymson RA, Will ME, Suter II GW, Wooten AC (1997). Toxicological Benchmarks for Screening Contaminants of Potential Concern for Effects on Terrestrial Plants: 1997 Revision. ES/ER/TM-85/R3. U.S. Department of Energy, Office of Environmental Management. 123p. |
[10] | Cal-EPA (2005). Air Toxics Hot Spots Program Risk Assessment Guidelines, Part II Technical Support Document for Describing Available Cancer Potency Factors. Office of Environmental Health Hazard Assessment, California Environmental Protection Agency. May 2005. |
[11] | Davidson EA, Belk E, Boone RD (1998). Soil water content and temperature as independent or confound factors controlling soil respiration in a temperate mixed hardwood forest. Global Change Biology 4: 217–227. |
[12] | Xu M, Qi Y (2001). Soil-surface CO2 efflux and its spatial and temporal variations in a young ponderosa pine plantation in northern California. Global Change Biology 7: 667–677. |
[13] | Turner BL, Haygarth PM (2001). Phosphorus solubilizationin rewetted soils. Nature 411: 258. |
[14] | Kanaly RA, Harayama S (2000) Biodegradation of High-molecular Weight Polycyclic Aromatic Hydrocarbons by Bacteria. J. Bacteriol., 182, 2059. |
[15] | Gharieb MM, Sayer JA, Gadd GM (1998). Solubilization of natural gypsum (CaSO4 _2H2O) and the formation of calcium oxalate by Aspergillus niger and Serpula himantioides. Mycol. Res. 102, 825-830. |
[16] | Nguyen MT (2006). The effect of temperature on the growth of the bacteria Escherichia coli DH5α. Saint Martin’s University Biology Journal, 1. Retrieved fromhttp://homepages.stmartin.edu/fac_staff/molney/website/SMU%20Bio%20Journal/Nguyen%202006.pdf |
[17] | Stetter KO (1998) Hyperthermophiles: isolation, classification, and properties. In: Horikoshi K, Grant WD (eds) Extremophiles: microbial life in extreme environments. Wiley-Liss, New York, pp 1–24 |
[18] | Müller R, Antranikian G, Maloney S, Sharp R (1998) Thermophilic degradation of environmental pollutants. In: Antranikian G (ed) Biotechnology of extremophiles. (Advances in Biochemical Engineering/Bio-technology, vol 61) Springer, Berlin Heidelberg New York, pp 155–169. |