Jared M. Ashcroft1, Ashley Min1, Isabel Bojanini1, Melanie Hacopian1, Kristine Schroeder2, Atilla O. Cakmak3, Brandon Rodriguez4
1Division of Natural Science, Pasadena City College, Pasadena, USA
2North Seattle College, Seattle Hub for Industry Driven Nanotechnology Education, Seattle, USA
3Nanotechnology Applications and Career Network, Pennsylvania State University, University Park, USA
4NASA Education, Jet Propulsion Laboratory, Pasadena, USA
Correspondence to: Jared M. Ashcroft, Division of Natural Science, Pasadena City College, Pasadena, USA.
Email: |  |
Copyright © 2018 Scientific & Academic Publishing. All Rights Reserved.
This work is licensed under the Creative Commons Attribution International License (CC BY).
http://creativecommons.org/licenses/by/4.0/

Abstract
In a series of activities/labs, designed in a building block approach, whereas each subsequent lab builds into the next, students will explore the reactivity and production of oxygen in various biological and chemical systems. Through student-constructed analyses, participants will optimize oxygen-generating systems for the colonization of Mars, wherein their system will be used as part of a narrative to construct a livable habitat for future astronauts. Use of remote access technology to a Scanning Electron Microscope (SEM) with elemental analysis capabilities allows students to investigate their oxygen reaction via formation of iron oxide, resulting in rich multidimensional and contextualized scientific exploration in the chemistry classroom.
Keywords:
Remotely Accessible Scanning Electron Microscope, Project-Based Learning, Chemical Reaction Analysis
Cite this paper: Jared M. Ashcroft, Ashley Min, Isabel Bojanini, Melanie Hacopian, Kristine Schroeder, Atilla O. Cakmak, Brandon Rodriguez, Cultivating Mars: A Project-Based Learning Lab Analyzing an Oxygen Based Redox Reaction in Order to Design an Oxygen-Rich Environment on the Red Planet, Journal of Laboratory Chemical Education, Vol. 6 No. 1, 2018, pp. 4-11. doi: 10.5923/j.jlce.20180601.02.
1. Introduction
Throughout history, exploration of the unknown has been a human quality. The list of momentous discoveries by explorers is endless. From the Viking Leif Ericson, first setting foot in North America 2000 years ago, to Sacagawea, guiding the Lewis and Clark expedition in 1804 shows these explorers came from all walks of life. In many instances, these explorers defeated the unknown; Jacques Cousteau probing under the sea, Edmund Hillary scaling the top of the world and Neil Armstrong walking on the moon. The human condition of conquering the impossible continues to this day in our aspiration to reach Mars. This is the next great exploration to overcome. NASA has established three phases to accomplish this endeavor; Earth Reliant, Proving Ground and Earth Independent [1]. This problem-based learning lab is formulated revolving around the third phase of Mars exploration, becoming Earth Independent. Specifically, an activity was developed that focuses on oxygen generation and analysis using simple, inexpensive experiments that can have effortless implementation into a high school classroom. The experiment will also utilize the Remotely Accessible Instruments in Nanotechnology (RAIN) Network, whose mission is to bring advanced technologies, such as scanning electron microscopes, throughout educational communities. Used in tandem, these experiments and tools will help student interest and passion for the sciences, as well as promote student success and equity in the science classroom.It is well established that oxygen is the most vital gas for the continuance of human existence. Our cells need a constant supply of oxygen in order to survive. Oxygen is transported through the body through hemoglobin and is delivered to the necessary organs and tissues in the body. It has been discovered that the Mars atmosphere consists mainly of carbon dioxide (95.97%) with only traces of oxygen gas (0.146%) [2]. Therefore, methods of making Mars habitable (Figure 1) have been investigated [3]. A major component of Mars habitation is formulating a process to develop and analyze oxygen.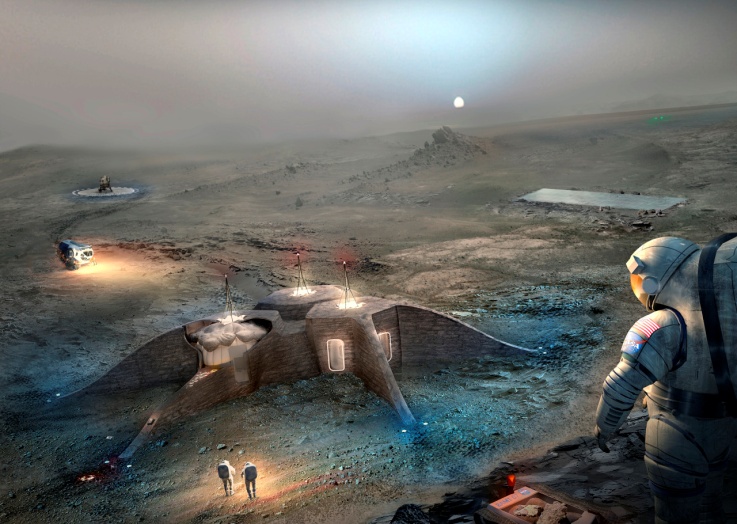 | Figure 1. A foreseen human habitat on Mars [4] |
The activity will proceed in modular fashion, whereas a series of activities will be performed in a systematic fashion, building upon each other towards a capstone project-based learning experience. First, a theoretical mass of oxygen for a room is calculated to demonstrate how significant figures play a role in experimental measurements, as well as demonstrate statistical and dimensional analysis. Second, a classic steel wool reaction with iron is performed, which leads to an experimental determination of percent oxygen that can be used to calculate the actual mass of oxygen in a room. Third, using a balance, Law of Conservation of Mass and empirical formula concepts can be explored by differentiating masses of steel wool before and after reaction with oxygen. Fourth, a remotely accessible Scanning Electron Microscope is utilized to image and obtain elemental analysis of the steel wool samples to confirm if the empirical formula for the iron oxide is valid. Finally, a project-based learning lab, developing a terrarium to optimize oxygen generation using plant life to support astronauts in space is explored.The activity begins by introducing students to the role that oxygen played in developing life on Earth using the short video “Photosynthesis Unleashes Chemical Superweapon: Oxygen” [5]. The video describes how photosynthesis from cyanobacteria allowed for a toxic chemical, oxygen, to invade early earth and describes how this chemical affected the creation of simple to multicellular organisms, up until the advent of Homo sapiens 200,000 years ago. The video is a precursor to introduce the experiment, measuring oxygen content in a chemistry classroom. Though the experiment is designed for high school or general chemistry course in college, preferably performed when discussing empirical formulas or chemical reactions, with some adjustments, a biology course could also benefit from this experiment. The last part of the experiment is optimal for an AP chemistry or biology class. The activity requires: 0.5 g steel wool, meter stick, 500 mL beaker, ring stand with test tube clamp, test tube, water, balance, laptop with projector acetone and 1 M acetic acid.Part 1: Determination of oxygen content in a room using theoretical percent oxygenThe activity begins with students determining the theoretical mass of oxygen in a room by measuring the room’s volume with a meter stick or ruler. It should be noted that several students will try and measure every desk, chair, etc. in the room. For comedy sakes, not telling them to assume the room is empty is preferred, but for logistical reasons, students should be told at the beginning of the activity to assume the room is empty. Once the measurements are concluded, the mass of oxygen in a room is calculated using known values of percent oxygen in air of 21% and density of air being 1.23 kg/m3. Each student in class performs the measurement, determines mass of oxygen in room in kg using dimensional analysis (see sample calculation below) and shares their results on the board. Table 1 is from measurements collected in a chemistry lab, which students converted to mass of oxygen in kilograms using dimensional analysis (Equation 1). The values were obtained from different students after measuring the volume of a room with a meter stick.Equation 1: Dimensional analysis determining mass O2 in room
Table 1. Dataset for mass of oxygen in a chemistry lab room  |
| |
|
Dimensional analysis would have been taught previously and this activity is a reinforcement of using this important technique. Significant figures and statistical analysis should have also been covered previously and sharing the data is a way to reintroduce the importance of determining significant figures experimentally, considering the precision of the measurement tool. In this case, using a meter stick to measure the volume of a room should allow for two significant figures. As shown in Table 1, not one student correctly determined significant figures using a meter stick. This is typical when performing this activity. It shows a disconnect students have with theoretical discussion of significant figures versus experimental determination. Once data is shared on the board, students are asked to write a conclusive statement about the mass of oxygen in the room as a take-home assignment. Surprisingly, 90% of the students will turn in a statement that uses only the individual data point they collected as the correct mass of oxygen in the room, neglecting to calculate the mean or standard deviation from the class data. Preferably, the mean and standard deviation would be determined and the mass of oxygen in the room for the above data would be 83 ± 7 kg O2 in room.Part 2: Experimental determination of percent oxygen and discussion of Part 1.Students are separated into groups of two. Each group of students are provided a small plastic box that contains steel wool, a 500 mL beaker, test tube, test tube clamp and a list of supplies, including water, ring stand, balance, acetone and 1 M acetic acid. A short discussion on acetone, detailing its high volatility and flammability should be performed. It can be mentioned that it is the main ingredient in fingernail polish remover and not toxic, but can cause a rash if spilled on skin or irritate the nose if smelled. Also mentioning that acetic acid is the main ingredient in vinegar, but should not be spilled on skin. Fingernail polish remover and vinegar can be used in cleaning the steel wool for the experiment if desired.A short article by Steve Riggs entitled “My Brain Needs Oxygen—What Can I Do?” [6] can be used to introduce this aspect of the experiment. This article inferred that a decrease of oxygen levels can have a negative impact on brain function. In this experiment, students must determine the percent of oxygen their classroom contains. Students must use the materials provided to determine the percent of oxygen in the room and convert this amount to the mass of oxygen that resides in the classroom as done in Part 1. This is an ideal opportunity to use a think, share, pair pedagogy for students to come up with possible methods of determining oxygen percent using steel wool and water. A subtle reminder to think about significant figures in actual measurements would be appropriate. Following their determination of oxygen content, they must plan a way to either decrease or increase the amount of oxygen in the room to optimize oxygen content. The ability to detail their methods for altering oxygen levels demonstrate an understanding of the task at hand thus far, serving as an excellent formative checkpoint and will also prepare them for Part 5 of the experiment.Percent oxygen is measured using established procedures [7]. Mass approximately 0.5 grams of steel wool and clean off the protective coating by dipping in acetone. Dry the steel wool with a paper towel and subsequently submerge the steel wool into the acetic acid solution. Again, dry off the acetic acid from steel wool and place in a test tube inverted over water (Figure 2a). Steel wool (iron) will react with water vapor in the test tube to form iron oxide (rust). Rusting will occur within 30 minutes and as oxygen is consumed, the water level in the test tube will rise, allowing for a measurement of oxygen in the test tube. Figure 2B displays a schematic of the experiment after oxygen consumption. Students may use a sharpie to mark the water level into the test tube to measure the distance water moved up the test tube, corresponding to oxygen displacement. Dividing the length water traveled up the test tube by the total length of test tube times 100 will give percent of oxygen in the room. An alternative method is possible wherein students measure total volume of water in the test tube, collect water through the reaction, use parafilm to remove the test tube from the water after reaction and measure volume of water that displaced air. Dividing this value by the total volume of water in test tube times 100 will give you percent oxygen in room.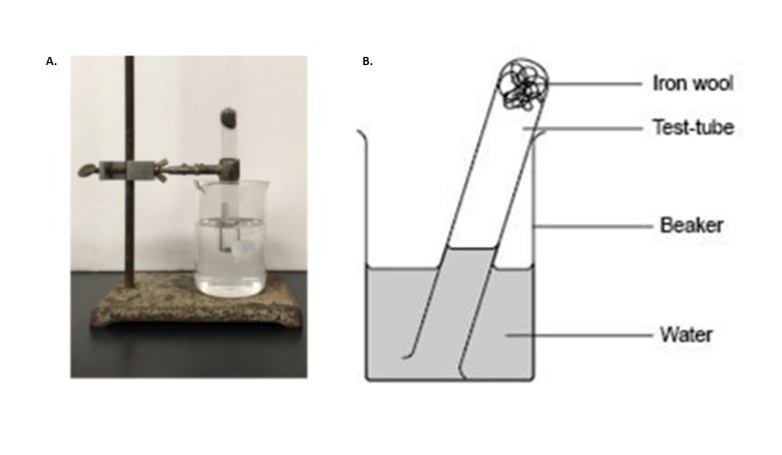 | Figure 2. A. Steel wool in test tube inverted over water. B. Schematic of water displacing air in test tube as oxygen is consumed |
As the experiment is in progress (takes between 15-30 minutes), a discussion from Part 1 can be done. Topics to discuss include the experimental determination of significant figures and the limit that measurement tools have on precision. For instance, mention that measuring volume of a room with a meter stick cannot have more than two significant figures. Discuss the value of using a set of data over an individual data point. It is worth mentioning at this point how sharing data as a class did bring in a variable of different students into the experiment and that will affect precision and accuracy of the measurement. A definition of standard deviation, a value that 90% of the actual measured values for the measurement reside in, can be explained. For instance, the values from the data set above show that 90% of the measured values reside between 76 and 90 kg. It is appropriate to also mention that outliers can be removed from data sets. For instance, the value 104.54 kg above is an obvious outlier that is acceptable to remove from the data set. For a more advanced class, it would be interesting to discuss how outliers are statistically removed using a t to q test.Students will once again share their data on the board (written as percent oxygen in room) and an assignment given to analyze the data and write a conclusion about the outcome of the experiment. At this point, the conclusion should be based on the class data and discuss why the experimental values of oxygen percent are lower or higher than the expected 21%. Typically, 80% of successful experiments show a percent oxygen level between 16 and 18 percent. Reasons for this low percent can be attributed to measuring oxygen content in a room that is constantly filled with people, consuming oxygen at a rate that is faster than the oxygen can be regenerated. Inherent errors in measurement techniques from the length and volumes of water in test tube can also factor into a decrease in percent oxygen. Rarely, a student will obtain an oxygen percent above the 21% expected value, which could be explained by excess water getting into test tube when transferring test tube in and out of water. Parts 1 and 2 can be assessed using the rubric in Table 2.Table 2. Rubric for determining oxygen content in a room 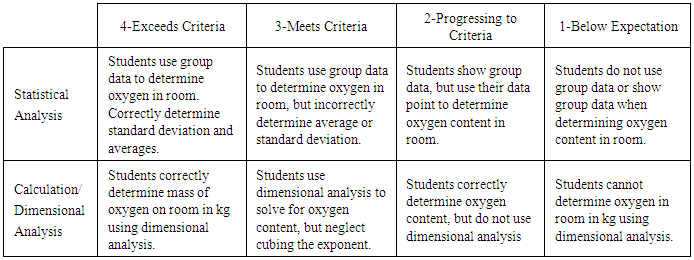 |
| |
|
Part 3: Empirical formula of steel wool and iron oxide using balance To determine the empirical formula of iron oxide after oxidation of steel wool, the mass of oxidized steel wool is subtracted from the mass of the original steel wool. This difference will be equivalent to the amount of oxygen in the oxidized steel wool sample. Using the mass of initial steel wool (iron) and the oxygen content, an empirical formula is determined using molar ratios calculated using mass to mole conversions. It should be noted that the empirical value determined using this method is dependent on the amount of steel wool used in the oxidation of iron. In a class of thirty high school students, not one was able to correctly identify the iron oxide as Fe2O3 using this method. This is due to the impracticality of getting an exact 2:3 molar ratio of iron to oxygen in the steel wool to oxygen reaction performed in Part 2 of the experiment.Part 4: Empirical formula of steel wool and iron oxide using RAIN The Remotely Accessible Instruments in Nanotechnology (RAIN) network is a conglomeration of eighteen colleges that provides free access to advanced scientific instruments through remote access from university laboratories to science classrooms [8]. It is easy to request a remote session at the RAIN Network website [9]. Instructions for arranging a RAIN session can be found in the classroom resources section of the website. Click on the Schedule a Remote Access Session button and choose a RAIN site from the list provided. For the percent oxygen lab, a Scanning Electron Microscope (SEM) is used to image the samples and an Energy Dispersive Spectrometer (EDS) is used to perform elemental analysis. Be sure to choose a site that has both of these instruments. Fill out the information and within 48 hours the RAIN network will make contact and start the planning phase for the remote session. These are free sessions and the only requirement is that at the end of the experience students and the instructor fill in the short, five-minute survey in the Getting Ready for Remote Access Step 6 section of the nano4me site. During the last year, over 50 remote sessions have been done with middle or high school campuses and only one instance occurred where the remote experience did not work. This was due to a misunderstanding in time zones. Therefore, it is advised to choose a RAIN site that is in your region. In all cases where a test session was done prior to the scheduled day, the remote session was a success and out of all K-12 teachers, all but one have said that it was a great experience and they would recommend a session to a colleague and do a remote session again. In order to perform remote access either Teamviewer or Zoom software, both of which are free, must be downloaded onto a computer attached to a projector. Samples that have been prepared at the RAIN host site of steel wool (Figure 3) and oxidized steel wool (Figure 4) will be imaged using the SEM and elemental analysis performed on each sample using EDS will give a mass percent of iron and oxygen in the two steel wool samples. These images and elemental analysis will be live streamed to your classroom and you can ask the RAIN technician questions about SEM or EDS theory. You can also access K-12 educator resources from the nano4me educator site. The remote session for imaging and analyzing steel wool will require 15 minutes of time. It can be arranged ahead of time for the RAIN site to show other images, such as spiders, butterfly wings, diatoms, etc. once the steel wool experiment has been completed. Though it is possible for students to control the instrument directly, it is advised that with a large class the RAIN technician controls the instrument while explaining the imaging. In sessions where one student controls the instrument, the other students have been bored and do not have a good experience.As shown in Figures 3 and 4, steel wool shows a mass percent of 100% iron, whereas the oxidized steel wool shows a mass percent of 69.5% iron and 30.5% oxygen. By assuming that we have 100 grams of steel wool, 69.5 g of iron and 30.5 g of oxygen are present in the oxidized steel wool sample and subsequent conversion to moles using the atomic mass of iron and oxygen leads to a molar ratio of 1.5 moles oxygen to 1.0 moles iron, giving an empirical formula Fe2O3, the most abundant iron oxide. A comparison of empirical formula determinations from mass calculations in part 5 and using the SEM demonstrates the advantage that advanced technologies have over conventional experimental methods. A rubric for empirical formula determination is included in Table 3.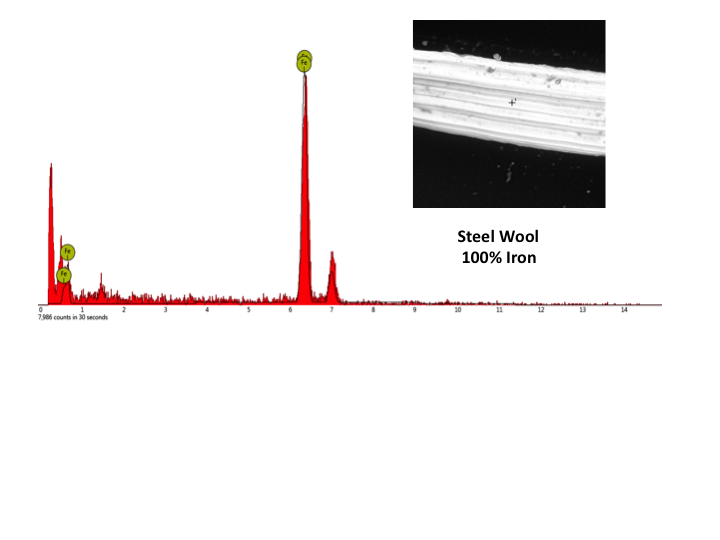 | Figure 3. SEM image and elemental analysis of steel wool |
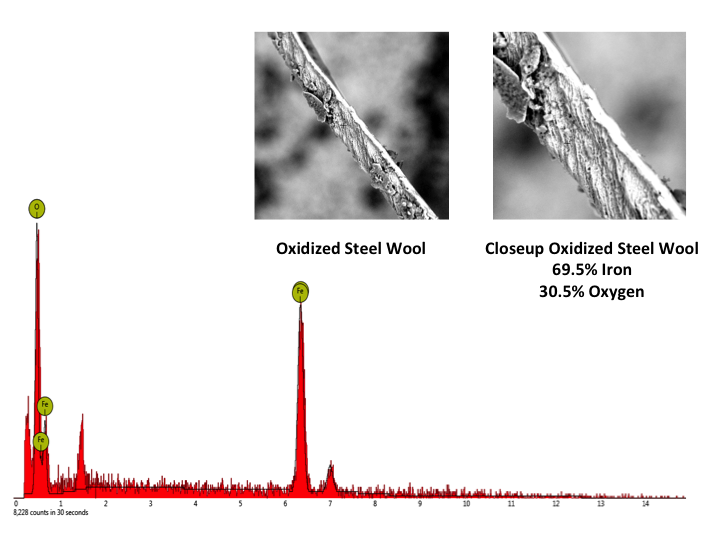 | Figure 4. SEM image and elemental analysis of oxidized steel wool |
Table 3. Rubric for empirical formula determination 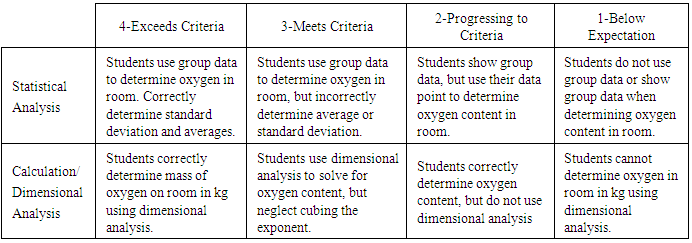 |
| |
|
Part 5: Project-based learning lab: Greenhouse for Mars This section of the experiment is designed to allow students to use their research and design skills to develop an oxygen producing system for use on a Mars habitat. Students will be assigned reading assignments “NASA May Put Greenhouse on Mars in 2021” [10] and “Designer Plants on Mars” [11]. From these reading assignments, students will be required to design a terrarium that could be used as a model for producing oxygen in a Mars habitat (See example Figure 5 and 6). The terrarium should contain oxygen-producing plants. An example of homemade terrariums can be found at http://inhabitat.com/how-to-make-your-own-terrarium/.This assignment should be done in groups, made at home and brought to campus after 3 weeks to see progress of plant growth and oxygen percent. During the three-week period, the terrariums should remain closed to the atmosphere. A second option is for students to make their terrarium at home and after three weeks make a video describing the terrarium, revealing the percent oxygen contained in the terrarium. The steel wool experiment should be done at the onset of terrarium construction and after the three-week period. In Figure 5A and 5B two terrariums made by different students with different plants and shapes were made. The terrarium in 5A had an oxygen content of 15% after three weeks, the same as when the terrarium was built. It should be noted the terrarium was placed outside during a heat wave where the temperature was over 100˚F for about two weeks leading to the plants dying.The terrarium in Figure 5B was constructed at the same time and showed an oxygen percent of 15% on day zero and after three weeks the oxygen percent in the terrarium increased to 25%. It is possible to see in Figure 5C the difference in oxidation of steel wool (test tube on the right) from terrarium 5B compared to the oxygen content in the room during the same time (test tube on the left). It was apparent from the image that the terrarium steel wool experiment demonstrated greater oxidation due to the brownish color and rusting of the steel wool in that test tube. 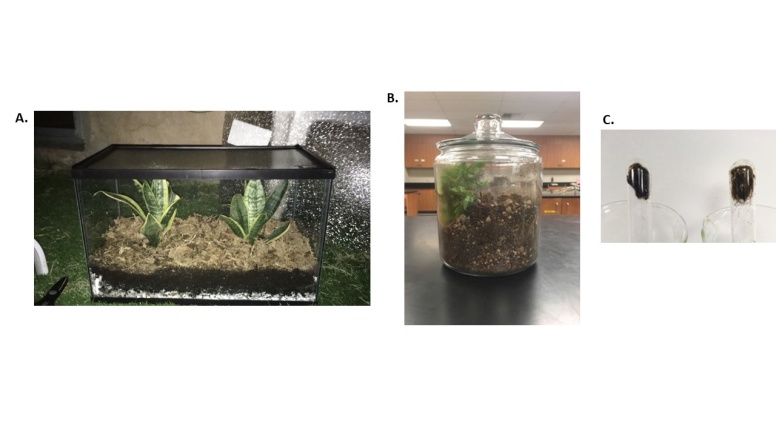 | Figure 5. A. Terrarium made from Sanseveria Superba (Snake Plant) and moss. B. Terrarium made from fern. C. Steel wool after oxidation right: from room left: from terrarium in B |
A third terrarium prepared by a student (Figure 6) contained plants existing in a water environment. Observation of the terrarium before (6A) and after three weeks (6B) shows a drastic difference of plant content in the terrarium. It should be noted that this terrarium when measured for oxygen content showed no increase from when the terrarium was first made. This could be due to not being able to put the test tube in the terrarium in order to measure oxygen content. Students should be told to add their test tube into the terrarium at the beginning of planting and keep the test tube in the terrarium during growth. One interesting aspect of terrarium in Figure 6C was the observation of “Pearling”, where the oxygen produced by plants in water becomes saturated causing streams of bubbles to generate in the water of the terrarium.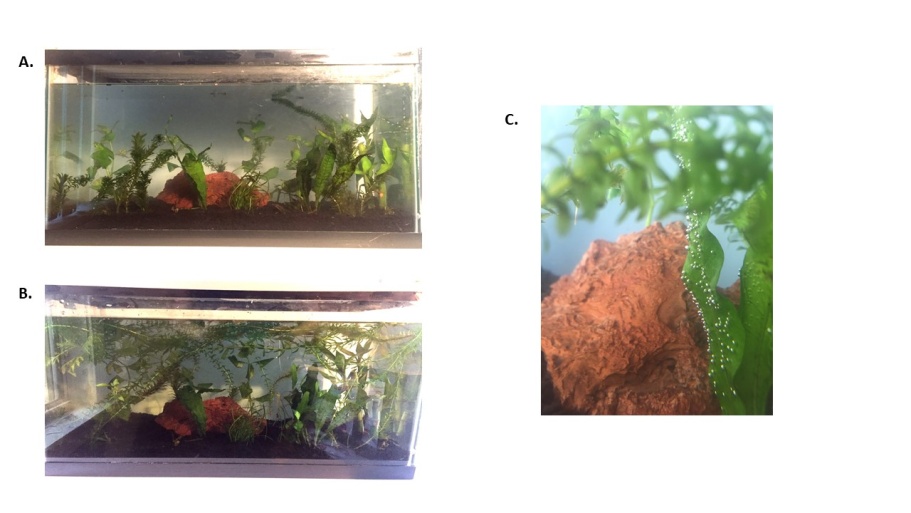 | Figure 6. A. Terrarium at day zero. B. Terrarium after 4 weeks. C. Oxygen generation from plants in terrarium (“Pearling”) |
The steel wool experiment is not an accurate method of determining percent oxygen in the air, but the goal should be to see a measurable increase in oxygen content in the terrarium after three weeks. At the end of the project, students present their terrarium to the class, as well as present a scaled-up design for a habitat of 4-6 astronauts living on Mars. Depending on where the teacher is in the curriculum, students can simply compare rates of consumption of oxygen by the astronauts versus generation by the plants to determine how big and how many plants their habitat will require. If their students are completing or have completed stoichiometry, students could convert between number of plants required per astronaut for a 12-month mission to Mars (a typical human consumes 550 L of oxygen per day). Classrooms can share their designed habitat online with other schools via the Imagine Mars resources provided by NASA [12].Table 4. Summary of steps for lab 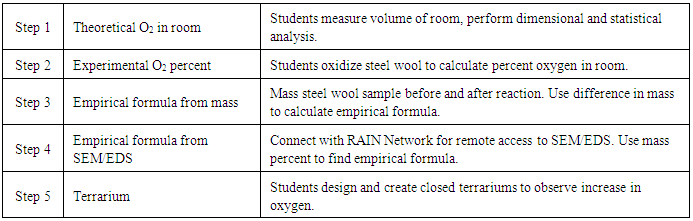 |
| |
|
2. Extensions
To combine the oxygen determination and terrarium build students could be asked to solve the following questions (terrarium B was used to solve the problems):1. How much oxygen in L is produced by your terrarium per day?
2. If astronauts need 550 L of oxygen per day, how big would the terrarium have to be to keep astronauts safe?The ratio of 550 L/day to 0.003 L/day terrarium is 1.8 x 105. Therefore 2 m3 times 1.8 x 105 equals a 3.7 x 105 m3 terrarium.3. If it takes 8 months to get from Earth to Mars, then how many liters of oxygen must we produce to supply a two-person crew?
4. Is using a terrarium a feasible method in supplying oxygen to astronauts in space? If not, write a short description of a method NASA is developing to supply astronauts with oxygen in space.No, the terrarium is not a feasible method of oxygen production for astronauts. NASA is developing the mars Oxygen ISRU Experiment (MOXIE) using a Scandia-stabalized zirconium electrolytic cell to convert CO2 to O2 on Mars.
3. Assessment
Students will create a design for a Mars habitat and write a lab report that should:1. Demonstrate ability to obtain a percent of oxygen using the oxidation of steel wool to iron oxide.2. Calculate the mass of oxygen in a room using dimensional analysis.3. Write a conclusion discussing what the percent and mass of oxygen in a room is. Students should be able to do statistical analysis on a set of class data, calculating average and standard deviation and in a conclusion, choose whether the individual or class data is best representative of oxygen content in the room.4. Determine the empirical formula of steel wool (iron) and rusted steel wool (iron oxide) using reactant and product masses as well as from the elemental analysis obtained from the RAIN Network. In a conclusion, students should discuss the advantages of using advanced technology to determine the empirical formula, using the data from the experiment to support the conclusion.5. Design a terrarium that utilizes various plants to optimize oxygen percent for a habitat on Mars and discuss the feasibility of using plants as an oxygen source in the colonization of Mars.The experiment allows for both formative and summative assessment. For formative assessment, students describe mechanisms for altering oxygen concentration early in the project, then near the conclusion present their Mars mission space design to the class and describe what types of plants and how they will best monitor oxygen content in their colony. Students are also required to write lab reports and work with one another, comparing experimental designs and be given feedback from peer-reviewed work. Summative assessments are also used to show students ability to solve dimensional analysis, empirical formula and statistical analysis problems based on the content in the lab, scaffolding up to stoichiometry, depending on when the activity is implemented in the curriculum. This summative assessment is performed as a lab practical, where students must demonstrate correct lab set up, as well as determine the percent of oxygen in room from and correctly perform averages and standard deviations from stations set up in the lab.
4. Teacher Feedback
This activity was initially demonstrated to 15 teachers at a problem-based learning workshop at Pasadena City College during Summer 2016. Teachers from schools that serve underrepresented students were selected for the workshop and were given supplies to perform the experiment in their class. Overall, the teachers gave positive feedback on the problem-based learning lab and enjoyed having access to an SEM and EDS for imaging and elemental analysis. During the process, teachers expressed a desire for the lab to contain a more project-oriented activity, leading to the development of Part 5 in the instructions. The full lab has been performed as described with students in two classrooms: a community college Introductory General Chemistry course, which is the equivalent of an AP chemistry course in high school, as well as in a traditional high school chemistry class. Students enjoyed the lab experience, but required more teacher instruction and class direction when performing the experiment. This is a common issue for students unfamiliar with problem-based learning projects, as students have difficulty adjusting to having the freedom to make choices and be allowed to make mistakes in order to enhance the learning experience. Several teachers discussed that having the open-ended, non-cookbook lab manual led to better discussion and learning. Additionally, teachers noted the ease of use performing the remotely accessible portion. It’s important to reiterate that the educators need to contact the RAIN Network and set up a test session in order to easily implement remote access to the advanced instrumentation. In some cases, where test sessions were not performed, difficulties arose in video and audio access to computers that made the remote session unattainable. It was suggested by all teachers to communicate fluidly with the RAIN host and to test the remote session before the day of the experiment.As a classroom management strategy, the first stage of the experiment where students invert the test tubes in the beakers of water does require 20-30 minutes to achieve best results. As such, when implemented it is best to start the lab, move on to some of the math activities or alternative material, then return to complete Part 2.
5. Conclusions
A chemistry experiment was developed to determine the percent of oxygen in air and used in tandem with remotely accessible instruments to determine the empirical formula of reactants and products in a chemical reaction. Statistical analysis was also performed on the data, as well as a cross disciplinary project in designing a terrarium that optimizes oxygen levels for a Mars habitat using plant life. These experiments align with the Next Generation Science Standards shown below. Using problem-based learning in conjunction with having accessible technologies available for students will benefit the understanding and excitement for science experimentation. Developing a series of problem-based learning lab modules to be used with the RAIN Network is an ongoing process that will be continually shared with educators across K-12, community college and four-year university classrooms.Standards AlignmentHS-PS1: Matter and its InteractionsPerformance expectations: Students will be able to describe and calculate a series of chemical proficiencies pertaining to dimensional analysis and simple chemical reactions. Students will also be able to engineer systems derived from their application of these chemical processes.HS-PS1-2: Construct and revise an explanation for the outcome of a simple chemical reaction based on the outermost electron states of atoms, trends in the periodic table, and knowledge of the patterns of chemical properties.Science and Engineering Practice:Constructing explanations and designing solutions in 9–12 builds on K–8 experiences and progresses to explanations and designs that are supported by multiple and independent student-generated sources of evidence consistent with scientific ideas, principles, and theories. Construct and revise an explanation based on valid and reliable evidence obtained from a variety of sources (including students’ own investigations, models, theories, simulations, peer review) and the assumption that theories and laws that describe the natural world operate today as they did in the past and will continue to do so in the future.Disciplinary Core Idea:PS1.B: Chemical Reactions: The fact that atoms are conserved, together with knowledge of the chemical properties of the elements involved, can be used to describe and predict chemical reactions.Crosscutting Concepts:Patterns: Different patterns may be observed at each of the scales at which a system is studied and can provide evidence for causality in explanations of phenomena. (HS-PS1-2), (HS-PS1-5)Stability and Change: Much of science deals with constructing explanations of how things change and how they remain stable. (HS-PS1-6)
ACKNOWLEDGEMENTS
The authors would like to thank the RAIN techs for assisting with the remote SEM portion of the lab. We would also like to the expertise of the NASA Educator Professional Development Collaborative. RAIN is a network supported by National Science Foundation grant DUE1204279. Melanie Hacopian and Isabel Bojanini are supported by BUILD PODER, funded by National Institute of General Medical Sciences of the National Institutes of Health under award number RL5GM118975.
References
[1] | NASA. “Journey to Mars Pioneering Next Steps in Space Exploration.” (October 2015): 1-35. |
[2] | Mahaffy, P. R., et al. “Abundance and Isotopic Composition of Gases in the Martian Atmosphere from the Curiosity Rover.” Science, 341, no. 6143 (2013): 263. |
[3] | McKay, C. P.; Toon, O. B and Kasting, J. F. “Making Mars Habitable.” Nature, 352, no. 6335 (1991): 489-496. |
[4] | NASA, “NASA Awards Top Three Design Finalist in 3_D Printed Habitat Challenge.” Last Modified September 2015. http://nasa.gov/directorates/spacetech/centennial_challenges/3DPHab/2015winners.html. |
[5] | The Science Channel, https://0x9.me/roFnQ, 2015. |
[6] | Riggs, S, “My Brain Needs Oxygen-What Should I Do?” http://www.nacd.org/my-brain-needs-oxygen-what-can-i-do/, NACD Foundation, Vol. 25, No. 5, 2012. |
[7] | Birk, J. P.; McGrath, L and Gunter, S. K. “A General Chemistry Experiment for the Determination of the Oxygen Content of Air.” Journal of Chemical Education 58, no. 10 (1984): 804-805. |
[8] | Ashcroft, J. M., et.al., “It’s RAINing: Remotely Accessible Instruments in Nanotechnology Promoting Student Success.” Current Issues in Emerging eLearning, Accepted August 2017. |
[9] | To schedule a remote session, visit the RAIN Network website at http://www.nano4me.org/remoteaccess. |
[10] | Wall, Mike. “NASA May Put Greenhouse on Mars in 2021.” https://www.space.com/25767-nasa-mars-greenhouse-rover-plant-experiment.html. 2014. |
[11] | Steigerwald, Bill. “Designer Plants on Mars.” https://www.nasa.gov/vision/universe/solarsystem/mars_plants.html. May 2005. |
[12] | NASA. “Mars Oxygen ISRU Experiment Project.” Last Modified 2014. http://Techport.nasa.gov. |