Alex R. Ketchum, Alexander K. Wolf, Mark E. Meyerhoff
Department of Chemistry, University of Michigan, Ann Arbor, USA
Correspondence to: Alex R. Ketchum, Department of Chemistry, University of Michigan, Ann Arbor, USA.
Email: |  |
Copyright © 2017 Scientific & Academic Publishing. All Rights Reserved.
This work is licensed under the Creative Commons Attribution International License (CC BY).
http://creativecommons.org/licenses/by/4.0/

Abstract
In the following experiment, organic chemistry students will gain hands-on experience with ultraviolet-visible and 1HNMR spectroscopy after synthesizing S-nitroso-N-acetyl-D-penicillamine (SNAP). SNAP is a popular small molecule nitric oxide (NO) donor that has shown promise for biomedical applications. Our group has consistently demonstrated that polymers, such as silicone rubber or polyurethanes, containing SNAP can release NO and use its potent antithrombotic and antimicrobial properties to prevent clot and bacteria biofilm formation. Following the straightforward acid catalyzed synthesis, students will characterize the product with 1HNMR. Studying SNAP’s 1HNMR spectrum exposes students to a variety of crucial concepts, including chemical shifts, integration, topicity, multiplicity, and coupling constants. Students can also calculate SNAP’s molar absorptivity using UV-Vis spectroscopy, and use it to monitor the compound’s stability in various environments. The experiment is presented in a manner most appropriate for an undergraduate, second-semester organic laboratory course; however, it can be readily tailored to first-semester students by omitting the 1HNMR portion. The stability tests are suitable for various environments and durations, allowing the experiment to be used for single or multiple class periods as desired.
Keywords:
Undergraduate organic chemistry experiment, S-nitrosothiol, 1HNMR spectroscopy, UV-Vis Spectroscopy
Cite this paper: Alex R. Ketchum, Alexander K. Wolf, Mark E. Meyerhoff, Synthesis, Spectroscopy, and Stability of the Nitric Oxide Donor S-nitroso-N-acetylpenicillamine: An Undergraduate Laboratory Experiment, Journal of Laboratory Chemical Education, Vol. 5 No. 4, 2017, pp. 79-85. doi: 10.5923/j.jlce.20170504.03.
1. Introduction
1HNMR spectroscopy is one of a synthetic chemist's most powerful and readily available tools for characterizing and studying a molecule’s structure and behavior. Additionally, UV-Vis spectroscopy is ubiquitous throughout many fields within the scientific community, ranging from organic, inorganic, analytical, materials science, and biochemistry. It can be used to identify molecules, observe reactions, and quantify molecular species [1-3]. Students with experience using these methods/instruments are likely to be well prepared and competitive for entering graduate school or starting an industrial position. In this undergraduate experiment, students will synthesize an S-nitrosothiol (RSNO), S-nitroso-N-acetylpenicillamine (SNAP), and use 1HNMR and UV-Vis spectroscopy to characterize and test its stability in various environments (Figure 1). RSNOs are a class of molecules containing a nitrosonium group bound to the sulfur on a thiol molecule. There are three known endogenous RSNOs, S-nitrosocysteine, S-nitrosoglutathione, and S-nitrosoalbumin, each of which functions as a nitric oxide (NO) donor in vivo [4]. In the 1980’s, scientists identified NO as the “endothelium derived relaxing factor,” due to the fact that it is released by the endothelium and promotes smooth muscle relaxation (controlling blood pressure). After this discovery, NO has been found to also have important antithrombotic and immunological roles, and serve as a signaling molecule for many biological functions [4-6]. Considerable research time and funding has been committed to thoroughly understanding and utilizing NO’s properties for medical applications [4, 5]. NO is unique in that it is a gaseous, radical-bearing molecule, which is the reason for much of its reactivity. NO is rapidly oxidized to nitrogen dioxide upon exposure to oxygen. Endogenously, it reacts with oxyhemoglobin to form methemoglobin. These reactions are responsible for NO’s brief half-life of a few seconds or less in blood. Consequently, scientists looking to use NO for medical applications currently study NO donors, such as SNAP, with the goal of creating stable and controllable NO release sources [4-8]. Although SNAP is to date one of the most stable RSNOs, it still is susceptible to decomposition during preparation and storage under some conditions. RSNOs release NO via thermal or photolytic activation—stimuli which are difficult to avoid (Reaction 1). Consequently, considerable research has focused on determining the stability of SNAP and other RSNOs in various environments so as to determine their suitability for biomedical applications [6, 9, 10].  | (1) |
In today’s world, health or medical applications are often at the forefront of scientific research. Areas such as medicinal chemistry, pharmaceutical science, and materials science are highly interdisciplinary and frequently involve synthetic, analytical, and spectroscopic techniques [1, 3, 11-13]. In this experiment, following the synthesis of this biologically-relevant molecule, students will perform analytical tests similar to those that are commonly used with SNAP or other NO donors during professional research [9, 10]. Students will nitrosate the thiol precursor, N-acetyl-D-pencillamine (NAP), in an aqueous/methanolic solution using acidified nitrite [6, 9, 10, 14, 15]. Reaction vessels should be covered in aluminum foil to minimize decomposition from exposure to light. Primary RSNOs are red in color; however, tertiary RSNOs, such as SNAP, are typically green. Consequently, students can visualize the nitrosation via the appearance of the green color (Fig. 1). In turn, as NO is released, SNAP decomposes into its corresponding disulfide, and loses its color, reverting to an off white solid (Fig. 2). After the reaction to prepare SNAP has reached completion, students can separate the SNAP crystals via vacuum filtration and confirm its identity via 1HNMR spectroscopy using d6-DMSO as the solvent. SNAP can be detected and the nitrosation conversion quantified using UV-Vis spectroscopy. RSNOs’ strong
absorptions between 320-360 nm allow straightforward and accurate measurements. SNAP’s molar absorptivity coefficient has been reported as ε340=1075 M-1cm-1 in water; however, this experiment provides an opportunity for students to learn about the Beer-Lambert Law (Eq. 1) by determining the molar absorptivity coefficient and using it to measure SNAP’s stability [9, 10, 14, 15]. The Beer-Lambert Law is fundamental to UV-Vis spectroscopy, thus making it a critical skill for students to master in their studies and eventually apply in almost any scientific research field. | (1) |
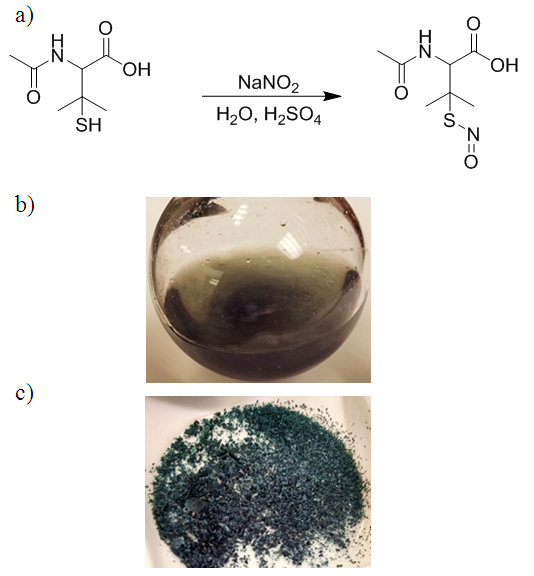 | Figure 1. a) Synthesis of S-nitroso-N-acetylpenicillamine (SNAP) via the nitrosation of N-acetylpenicillamine using acidified nitrite. b) Students can visualize nitrosation via the appearance of SNAP’s characteristic green color. c) SNAP crystals following vacuum filtration |
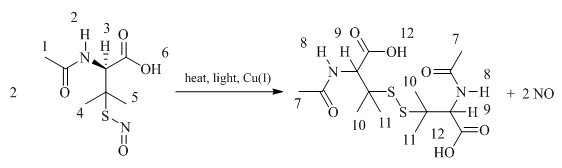 | Figure 2. SNAP decomposes to form the corresponding disulfide (RSSR) and NO. Positions are numbered as references for Figures 3, 4, 5, and Table 1 |
2. Experimental
2.1. Materials and Methods
N-acetyl-D-penicillamine, deuterated dimethyl sulfoxide, sodium nitrite, ethylenediaminetetraacetic acid (EDTA), potassium chloride, sodium phosphate dibasic, potassium phosphate monobasic, and methanol were purchased from Sigma-Aldrich (St. Louis, MO). Concentrated hydrochloric and sulfuric acids were products of Fisher Scientific (Pittsburgh, PA). Aluminum foil was purchased from Meijer (Ann Arbor, MI). Calculations were performed using Microsoft Excel (version 10). UV-Vis measurements were obtained using a Lambda 35 UV-Vis spectrophotometer (Perkin-Elmer, MA). 1HNMR spectroscopy was performed using a Varian 400 MHz spectrometer and the data processed using MestReNova. pH 7.4 phosphate buffered saline (PBS), containing 10 mM sodium phosphate, 138 mM NaCl, 2.7 mM KCl, and 100 µM EDTA was prepared using DI water. Depending on the experience level of the students, the PBS buffer and acidic stock solutions can be prepared in advance by staff. All reagents used are relatively inexpensive, further facilitating the adaptation of this experiment for an undergraduate lab class.Safety Notes to Instructors1. Students should practice standard safety precautions at all times. Students must wear safety goggles, gloves, and minimize exposed skin (only wear closed toe shoes, etc.). Synthesis and purification techniques, as well as sample preparations, should be performed in a fume hood behind a sash to prevent inhalation of organic solvents, and decrease the risk of students having direct skin contact with the reaction solution via splashing. 2. Hydrochloric and sulfuric acids are very hazardous, and pose the greatest risk for this experiment. Skin and eye contact, ingestion, and inhalation must be avoided. Risk levels can be reduced if staff prepares the acidic stock solution in advance. Care must be taken to add acid to water, not vice versa. 3. SNAP may cause skin, eye, and respiratory irritation, but poses negligible risk if standard safety precautions are followed. Storing the vials containing SNAP outside of the fume hood poses no additional danger since solutions are made with PBS buffer.
2.2. SNAP Synthesis
SNAP was synthesized using a modified version of previously reported methods [6, 9, 10, 13-15]. 25 mL of 1.0 M HCl(aq) solution containing 1 mL of conc. H2SO4 was prepared and poured into a 50 mL RB flask containing 1.0 g NAP (5.2 mmoles) and a stir-bar. Following 5 min vigorous stirring, the flask was covered with aluminum foil and charged with 0.71 g NaNO2 dissolved in 5 mL DI water over the course of 5 min. The aluminum foil can be briefly peeled back to observe the green color change corresponding with the synthesis of SNAP. After 45 min of stirring, the solution was chilled on ice and the SNAP crystals were isolated via vacuum filtration. The crystals were then rinsed with ice cold water to remove salt byproducts and then subsequently with acetone previously chilled in an ice bath [6, 9, 10, 14, 15]. Finally, the crystals were rinsed with diethyl ether to facilitate drying. The SNAP crystals were scraped into a beaker and spread out to maximize surface area before drying under a low stream of N2. SNAP can also be dried via rotary evaporation; however, students will likely need to be monitored initially to prevent the solvent from “bumping”. Crystals were weighed to determine yield, taking care to minimize light exposure.
2.3. Proton Magnetic Resonance (1HNMR) Spectroscopy
Spectra were obtained in d6-DMSO and shifts identified were referenced to DMSO’s resonance at 2.5 ppb. Peaks were picked, coupling constants measured, and the integration determined.
2.4. Molar Absorptivity
SNAP’s molar absorptivity was determined following synthesis. Solutions of 0.80, 0.50, and 0.25 mM of SNAP in PBS buffer were prepared for UV-Vis measurements. Students should be sparing when making samples to conserve SNAP for subsequent spectroscopic measurements. After testing each sample, a calibration curve was obtained via Microsoft Excel using the absorbance values at 340 nm wavelength for each solution. The 0.80 mM SNAP solution was used for further stability studies.
2.5. Stability Studies
The 0.80 mM SNAP(PBS) samples were prepared in 20 mL vials using PBS buffer with EDTA present and exposed to varying degrees of heat and light. The vials should contain an adequate volume of SNAP(PBS) for UV-Vis measurements, as determined by the cuvette size. An excess is optimal to compensate for any volume lost during transfers. Exposure conditions can vary and be tailored to the number of students, and access to available light sources, e.g. windows, fluorescent lights, etc. Samples were placed on a window sill and in a hood, cupboard, and refrigerator [10] to provide a variety of thermal and light exposures. Some samples were also stored in a dark 37°C oven to simulate physiological conditions. Each sample’s RSNO concentration was tested via UV-Vis spectroscopy at regular intervals. The heated vials were briefly placed in a refrigerator to reduce the solutions’ temperatures to approximately RT to minimize evaporation during measurements. The vials were immediately returned to their appropriate storage conditions afterward. Absorbances at 340 nm were recorded and used to calculate remaining SNAP levels, which in turn, plotted over time to exhibit the varying effects of the stimuli [10]. The time intervals are flexible and can be modified to allow for variation in class duration and frequencies.
3. Results and Discussion
3.1. SNAP Synthesis
Although nitrosation is commonly a quantitative reaction, the workup causes some product to be lost in the process due to SNAP’s partial solubility in acetone. We obtained 54 ± 7% yields for n=3. Assuming the procedure is accurately followed, and the molar absorptivities are calculated soon following the synthesis (to minimize sample decomposition), students should obtain molar extinction coefficients close to the reported value, ε340=1075 M-1cm-1 [9]. If instrumentation is limited, SNAP samples can be stored in a cupboard or refrigerator to prolong lifetimes. Since the reaction is reliably reproducible, some students can determine the molar absorptivity prior to performing 1HNMR spectroscopy, if necessary. As long as samples are stored in the dark at or below room temperature, negligible decomposition will occur during the processes [10].
3.2. 1HNMR Spectrometry
SNAP’s 1HNMR spectrum was taken following synthesis and is displayed in Figure 3a. The peaks are numbered as shown in Figure 2, with the corresponding proton shifts detailed in Table 1. At this point minimal disulfide is present, allowing easy and accurate characterization. SNAP’s synthesis is easily integrated within undergraduate curriculum as the reaction is reliably quantitative, and consequently has minimal residual starting material that could otherwise convolute the spectra [9, 10, 14]. There is minimal overlap of shifts and the integration aligns well between SNAP’s protons. The N-H and adjacent C-H protons at positions 2 and 3, respectively, are distinct, and this serves as an opportunity for students to determine the coupling constants. These protons also work superbly for comparing SNAP:RSSR concentrations during decomposition, as they too are distinct (Fig. 4). Due to inherent chirality, the protons at positions 4 and 5 in SNAP and 10 and 11 in the corresponding disulfide are diastereotopic. The resulting upfield resonances are useful traits for teaching students about topicity (See Figure 5b).Table 1. Proton shifts, splitting, and coupling constants for SNAP (1-6), and the RSSR (7-12) 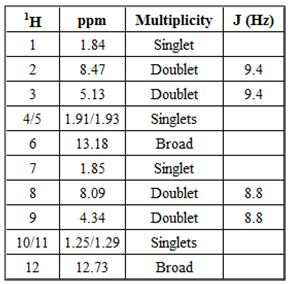 |
| |
|
An intermediate spectrum was obtained following partial SNAP decomposition so as to demonstrate the SNAP:RSSR proton resonances relative to each other. The amide and neighboring C-H proton shifts can be integrated to compare concentrations. RSNOs’ 2:1 stoichiometric decomposition must be taken into account when comparing the relative composition of each species. Protons at positions 3 and 9 serve as integration comparisons due to their aprotic nature. In Figure 4b, 0.54/(0.54+1.00/2) = 52% of SNAP remains. If time is available, students can take spectra during and/or following decomposition to compare SNAP and the dimer’s coupling constants: 9.4 and 8.8 Hz, respectively. Finally, Figures 3c, 4c, and 5 display the dimer following decomposition.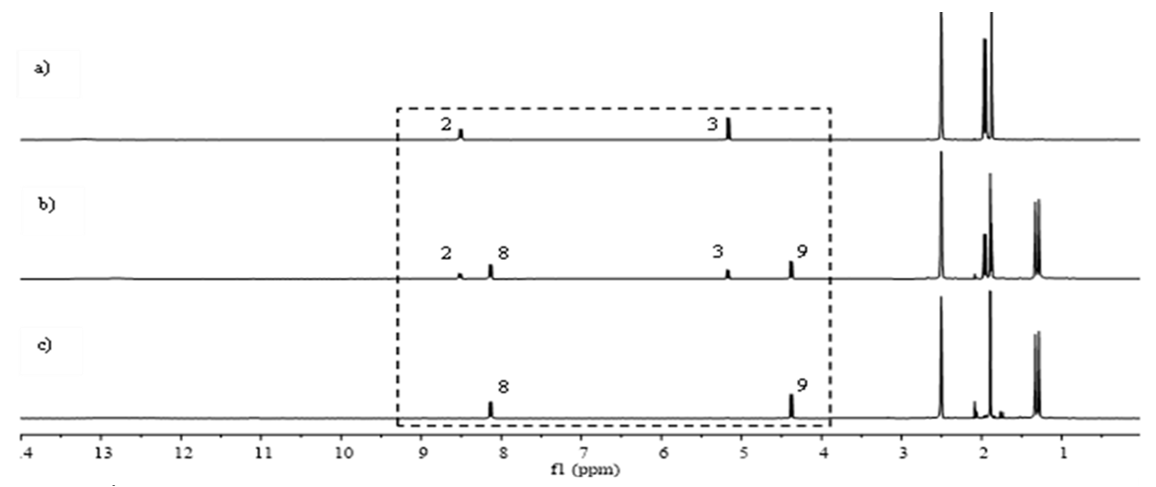 | Figure 3. 1HNMR spectra corresponding to a) pure SNAP, b) partially decomposed SNAP, and the c) disulfide decomposition product. Samples were dissolved and tested in d6-DMSO on a 400 MHz Varian NMR spectrometer |
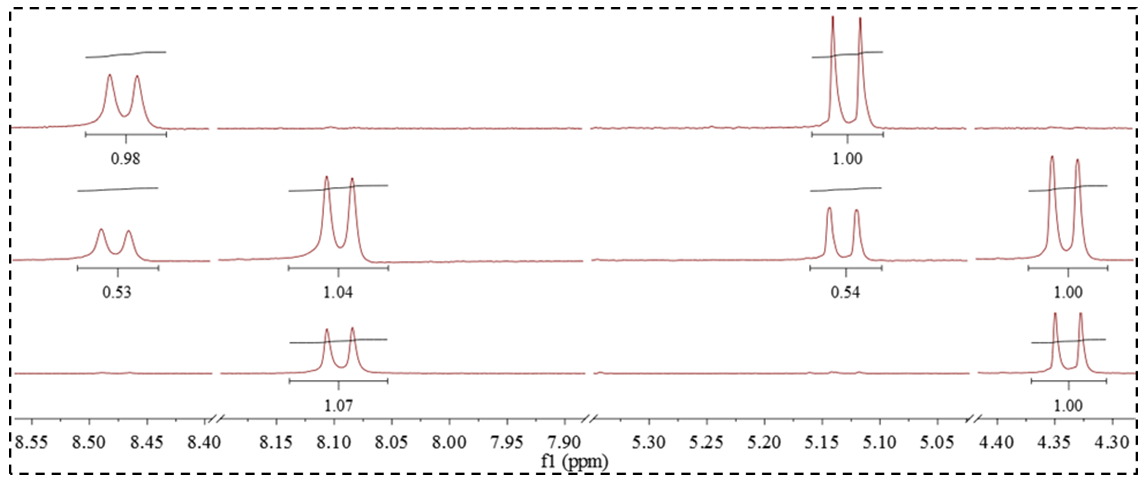 | Figure 4. Close-up of the pair of doublets corresponding to SNAP’s N-H and C-H at positions 2 and 3, respectively, as well as the corresponding disulfide protons at positions 8 and 9. Integration is referenced to the C-H at position 3 and 9 of SNAP and the disulfide, respectively. Spectra correspond with a) pure SNAP, b) partially decomposed SNAP, and the c) disulfide product |
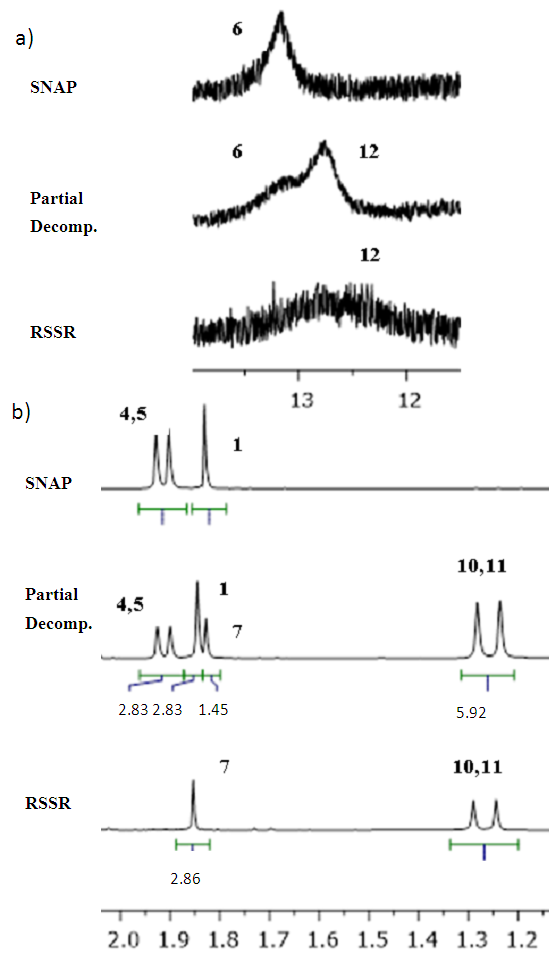 | Figure 5. Expanded region showing SNAP and the disulfide’s a) carboxylic acid b) methyl protons. Each region shows shifts from samples containing pure SNAP, the mixture following partial decomposition, and pure RSSR |
3.3. UV-Vis Spectroscopy
RSNOs absorb light in the visible region of the spectrum; however, this absorption is considerably weaker than typically observed for organic molecules in the ultraviolet region. Nonetheless, we determined SNAP’s molar absorptivities to be ε340=1079 M-1cm-1 and ε591=17.3 M-1cm-1. This agrees well with the recently reported value, ε340=1075 M-1cm-1 [9, 10]. The corresponding spectra and calibration curves are shown in Fig. 6.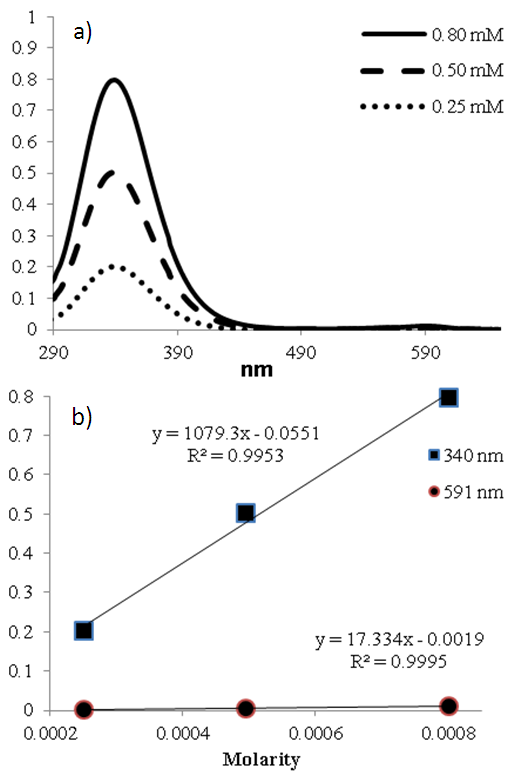 | Figure 6. a) UV-Vis spectrum of SNAP at 3 different concentrations. The absorbance at 340 nm was subsequently used to determine the molar absorptivity as shown in the calibration curve (b) |
3.4. Stability
We tested the SNAP solutions using UV-Vis spectroscopy for 7 days in a range of different conditions (Figs. 7 and 8); however, this variety is not required for a successful experiment. The most useful conditions for drawing comparisons would be those separately displaying the effects of temperature and light; i.e. a combination of available dark environments: covered vial in a cupboard/refrigerator/oven, and separately: windowsill/hood/cupboard, to observe the effects of light. It is worth noting that the observed stabilities are circumstantially dependent, and the students’ SNAP concentrations may differ from ours due to factors such as room temperature, distance from windows, and cloud cover. The significance lies in the results relative to one another. Photolytic decomposition can be observed in as little as 30 min, with 23, 9, and 5% SNAP lost for samples stored on a window sill, in a lab hood, and in a cupboard, respectively. The rapid photolytic decomposition allows the experiment to be concluded within a single class period, if desired. The effects of light on NO release were apparent during subsequent measurements as well. After 3 h, 13% of SNAP had decomposed for samples at room temperature that were not exposed to the light (cupboard), compared to 23% with the samples in the hood. SNAP solutions exposed to direct sunlight had lost 40% by the 3 h timepoint. The samples with direct light exposure had fully decomposed to the disulfide in less than 2 days. Students should readily be able to conclude that light exposure stimulates NO release.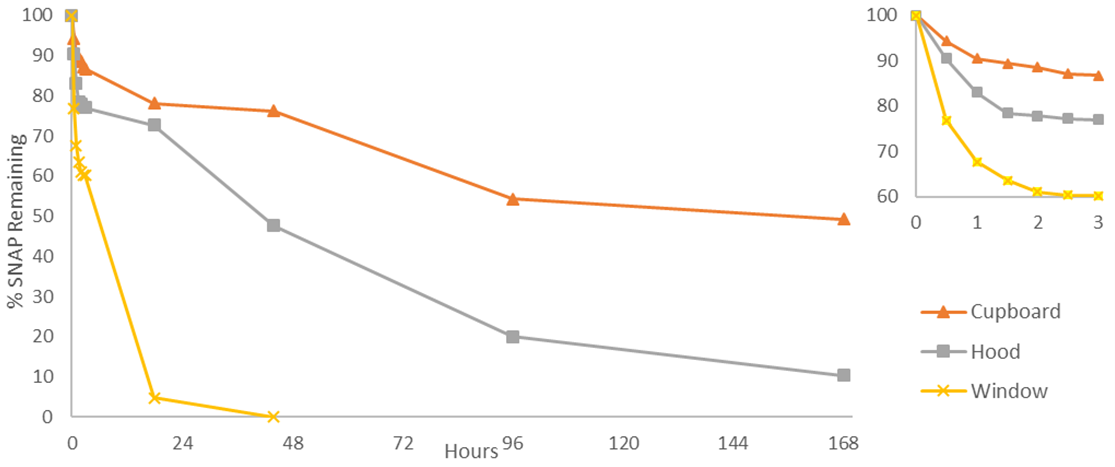 | Figure 7. 0.8 mM SNAP(aq) solutions exposed to varying degrees of light while stored in 3 different locations. The inset shows the initial 3 h expanded. The % of remaining SNAP was calculated using SNAP’s UV absorbance at 340 nm |
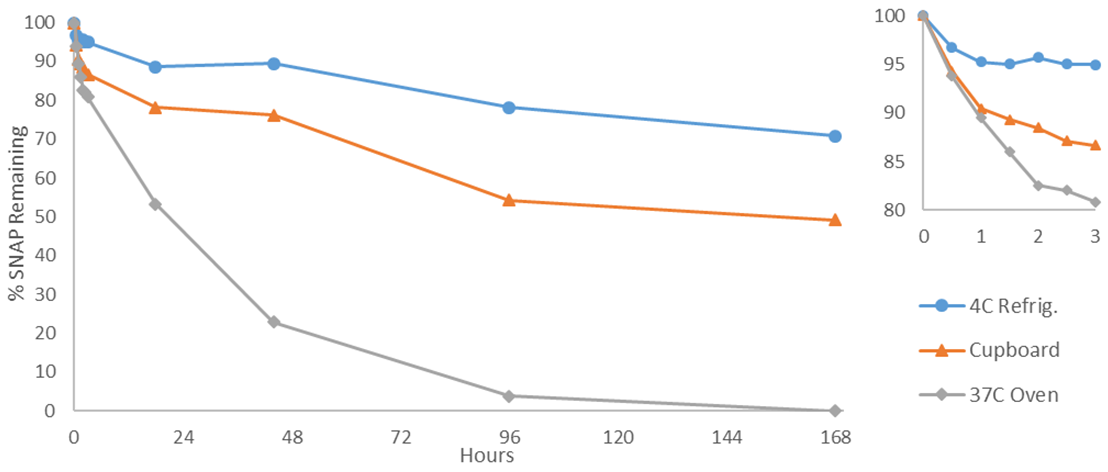 | Figure 8. 0.8 mM SNAP(aq) solutions exposed to varying degrees of heat while stored in 3 different locations. The inset shows the initial 3 h expanded. The % of remaining SNAP was calculated using SNAP’s UV absorbance at 340 nm |
SNAP is more stable with regard to the tested temperature range (Fig. 8). 6% of the SNAP decomposed from the 37°C samples after 30 min., while 5 and 3% were lost from the cupboard and refrigerator, respectively. After 3 h, 81, 87, and 95% SNAP remained in the oven, cupboard, refrigerator, respectively. The distinct SNAP concentrations should facilitate the students’ abilities to draw a conclusion regarding the effects of temperature on NO release. The oven samples had released nearly all the NO after 4 days (4% SNAP remained), whereas the cupboard and refrigerator samples contained 49 and 71% SNAP, respectively.The experiment can easily be extended to 2 lab periods, if desired, by measuring additional data points and optionally monitoring the decomposition with 1HNMR. This may be helpful if equipment is limited or class sizes are large and minimal time is left for stability testing following characterization.
4. Conclusions
The experiment described herein provides an opportunity for undergraduate chemistry students to develop and strengthen several crucial skills that are applicable in many scientific fields [1-3, 9-11]. Students will gain valuable experience using a UV-Vis spectrophotometer, and learn about the Beer-Lambert Law by determining SNAP’s molar absorptivity. In d6-DMSO, SNAP’s structure is inherently useful for gaining experience with 1HNMR spectroscopy. In addition to measuring SNAP’s proton resonances and integrations, students can investigate spectroscopic topics including multiplicity and coupling constants. Testing the molecule’s stability places students within a model that closely simulates industrial and academic chemistry research, as they witness firsthand the relevance of stability for potential products [9, 10]. Indeed, SNAP and other RSNOs are being examined as NO donors to develop a new generation of thromboresistant and antimicrobial intravascular and urinary catheters, etc. [9, 10]. RSNOs are rarely included, and thus critically underutilized, in undergraduate organic lectures and laboratories despite their popularity and significance in current biomedical research. This experiment is applicable in a wide range of laboratory settings, allowing variation for the number of students, experience levels, desired experimental duration, and available instrumentation. Due to SNAP’s useful properties, it provides a simple addition to any curriculum to expand upon thiol or disulfide chemistry, while allowing students to practice using two of the most important scientific instruments in chemistry [1-3].
ACKNOWLEDGEMENTS
We thank the NIH (grants EB023294 and HL128337) for supporting research in our laboratory relating to use of SNAP to develop new biomedical devices.
References
[1] | A. Rahman, M. I. Choudhary, Structure-activity relationship studies in drug development by NMR spectroscopy, Sharjah, United Arab Emirates: Bentham Science Publishers, 2011, vol. 1. |
[2] | J. W. Robinson, E. S. Frame, G. M. Frame II, Undergraduate instrumental analysis, Boca Raton, USA: CRC Press, 2014, 7 Ed. |
[3] | A. L. Skinner, J. S. Laurence, “High-field solution NMR spectroscopy as a tool for assessing protein interactions with small molecule ligands,” J. Pharm. Sci., 2008, 97, pp. 4670-4695. |
[4] | H. H. Al-Sa-doni, A. Ferro, “S-Nitrosothiols as nitric oxide-donors: chemistry, biology and possible future therapeutic applications,” Curr. Med. Chem., 2004, 11, 2679-2690. |
[5] | N. Tuteja, M. Chandra, R. Tuteja, M. K. Misra, “Nitric oxide as a unique bioactive signaling messenger in physiology and pathophysiology,” J. Biomed. Biotechnol., 2004, 4, 227-237. |
[6] | I. Chipinda, R. H. Simoyi, “Formation and stability of a nitric oxide donor: S-nitroso-N-acetylpenicillamine,” J. Phys. Chem. B., 2006, 110, 5052-5061. |
[7] | A. J. Gow, B. P. Luchsinger, J. R. Pawloski, D. J. Singel, J. S. Stamler, “The oxyhemoglobin reaction of nitric oxide,” Proc. Natl. Acad. Sci., 1999, 96, 9027-9032. |
[8] | U. Schedin, C. G. Frostell, L. E. Gustafsson, “ Formation of nitrogen dioxide from nitric oxide and their measurement in clinically relevant circumstances,” Br. J. of Anaeseth., 1999, 82, 182-192. |
[9] | A. Colletta, J. Wu, Y. Wo, M. Kappler, H. Chen, C. Xi, “S-Nitroso-N-acetylpenicillamine (SNAP) impregnated silicone Foley catheters: a potential biomaterial device to prevent catheter associated urinary tract infections,” ACS Biomater. Sci. Eng., 2015, 1, 416-424. |
[10] | E. J. Brisbois, H. Handa, T. C. Major, R. H. Bartlett, M. E. Meyerhoff, “Long-term nitric oxide release and elevated temperature stability with S-nitroso-N-acetylpenicillamine (SNAP)-doped Elast-eon E2As polymer,” Biomaterials, 2013, 34, 6954-6966. |
[11] | A. F. Repko, Interdisciplinary research: process and theory, Thousand Oaks, USA: SAGE, 2008. |
[12] | http://benefunder.org/causes/501/debbie-crans; accessed Aug 10, 2015. |
[13] | L. S. Choi, H. Bayley, “S-Nitrosothiol chemistry at the single-molecule level,” Angewandte Chemie – Internat. Ed., 2012, 51, 7972-7976. |
[14] | D. L. H. Williams, Nitrosation reactions and the chemistry of nitric oxide, Amsterdam, Netherlands: Elsevier, 2004. |
[15] | C. W. McCarthy, J. Goldman, M. C. Frost, “Synthesis and Characterization of the Novel Nitric Oxide (NO) Donating Compound, S-Nitroso-N-acetyl-D-penicillamine Derivatized Cyclam (SNAP-Cyclam),” ACS Appl. Mater. Interfaces, 2016, 8, 5898-5905. |