Mousaab M. Nahas
Electrical and Computer Engineering, Faculty of Engineering – North Jeddah, King Abdulaziz University, KSA
Correspondence to: Mousaab M. Nahas , Electrical and Computer Engineering, Faculty of Engineering – North Jeddah, King Abdulaziz University, KSA.
Email: |  |
Copyright © 2012 The Author(s). Published by Scientific & Academic Publishing.
This work is licensed under the Creative Commons Attribution International License (CC BY).
http://creativecommons.org/licenses/by/4.0/

Abstract
In this paper, we present 50 GHz spaced 25
40 Gbit/s WDM transmission over 560 km using SMF-based Large Effective Area Fiber (LEAF) in a recirculating loop. The paper uses band-limited RZ signals carrying 231-1 PRBS data, and shows that transmission distance of 560 km can be achieved with BER ≤ 10-9 using 1 mW peak power and 4 ps pulse-width for each data signal. To attain this, optical filters with sharp transmission characteristics are used in both transmitter and receiver. The results demonstrated in this paper are based on simulation, and the author believes that the propagation distance reached in the paper is the longest distance achieved for such system
Keywords:
Optical Fiber Communication, Dense Wavelength Division Multiplexing (DWDM), Optical Time Division Multiplexing (OTDM), High Speed Optical Transmission
Cite this paper: Mousaab M. Nahas , 50 GHz Spaced 25 × 40 Gbit/s WDM Transmission Over 560 km Using SMF-Based Large Effective Area Fiber (LEAF), International Journal of Optoelectronic Engineering, Vol. 2 No. 1, 2012, pp. 17-20. doi: 10.5923/j.ijoe.20120201.04.
1. Introduction
Increasing the transmission capacities of optical fiber communication systems has been a big demand since these systems were first developed. In fact, increasing the capacities is still under development as telecommunications keep expanding over time. It is well known by optical communication people that increasing the capacity of optical fiber systems can be either achieved through wavelength division mutliplexing (WDM) or optical time division multiplexing (OTDM) or by a combination of both. OTDM is more economic in network operation as the number of terminals is reduced and also it can accommodate the existing single-band and narrow-band erbium-doped fiber amplifiers (EDFA’s) without a need to replace them by broadband amplifiers. Considering this, it would be more convenient in many cases to generate single 40 Gbit/s signal through OTDM rather than using 4
10 Gbit/s WDM signal. As well, this 40 Gbit/s capacity can be multiplied through a combination of multiple 40 Gbit/s signals using WDM thus the capacity increases significantly. This OTDM-WDM combination approach is commonly used in high speed optical transmission systems[1]-[3]. However, since most existing (legacy) fiber optic networks are based on single mode fiber (SMF), it is still much useful to investigate and upgrade systems using this conventional fiber in their transmission channels although longer distances can be achieved using other types of fibers as presented in[4], which used dispersion shifted fiber (DSF) in soliton. In reality, the most worldwide deployed SMF fibers are: standard single mode fiber (SSMF); and large effective area fiber (LEAF). A lot of investigation has been done on multiple 40 Gbit/s signals using SSMF as presented in[2], in which 511 km propagation distance was reached using NRZ modulation format. Another investigation on SSMF was demonstrated in[3] showing 4
40 Gbit/s WDM transmission over 300 km using RZ format. In practice, the other fiber type (LEAF) has shown better results in different modulation formats due to reduced nonlinear effects in the fiber during propagation. Good results are presented in[5] using dubinary signals over LEAF. In terms of modulation format, it has been proven that RZ signals are more reliable than NRZ for long-haul transmission and most common in conventional systems using OTDM[6]. Based on this argument, this paper is concentrating on transmitting multiple 40 Gbit/s signals over LEAF using band-limited RZ signals. In such regime, an investigation should involve exploring the optimum peak power and pulse-width of the transmitted signals so that the longest possible transmission distance is achieved with acceptable error rate. Indeed, these two parameters play the major role as peak power can cause nonlinearities while pulse-width can lead to distortion due to polarization mode dispersion (PMD) within the fiber. This kind of study was already presented in[7] showing good transmission results for single 40 Gbit/s channel over 1100 km of LEAF using 7 mW signal peak power and 8 ps band-limited RZ Signals. Further investigation has shown successful transmission of 50 GHz Spaced 4
40 Gbit/s WDM signal over 700 km using 3 mW peak power and 6 ps pulse-width[8]. Larger capacity transmission over less propagation distance was demonstrated earlier in[9], where experimental results of 25
40 Gbit/s DWDM transmission over 480 km was presented using 10 ps RZ signals. However, that experiment did not investigate different pulse-widths other than 10 ps thus no one is certain whether this pulse-width is optimal for the system presented. Also, in[8], it was believed that simulating a few DWDM signals is somehow sufficient to predict the behavior of systems carrying higher number of signals while using small CPU time. Therefore, it was preferred to optimize the propagation distance at the expense of the capacity. In this paper, we believe that it is still more beneficial to examine larger number of signals (channels) on the same system in order to better simulate real high capacity DWDM systems though the CPU time multiplies considerably. On the top of that, this paper uses 231-1 data length in all channels which is much longer than the bit streams used in[8] and[9].
2. Experimental Setup
The setup diagram of our experiment is shown in Figure 1. At transmitter, twenty five laser diodes are used with wavelengths ranging from 1545.6 nm to 1555.2 nm using 50 GHz spacing. The twenty five wavelengths are DWDM multiplexed and then modulated by 10 Gbit/s band-limited RZ data signal to give 25
10 Gbit/s signals. The data pattern used is PRBS with 231-1; 50% ones. A 25
40 Gbit/s bit stream is then generated through two stages co-polarized OTDM, as depicted in the setup diagram. A fiber link of 800 km is composed using 20
40 km recirculating loop consisting of 2
10 km SMF-based Large Effective Area Fiber (LEAF) with positive dispersion, one 20 km dispersion slope compensating fiber (SCF) and one broadband erbium-doped fiber amplifier (EDFA) repeater. This SMF-SCF configuration allows dispersion flattening over the fiber span within the loop with reduced intra-span dispersion excursion[9]. The dispersion, dispersion slope and effective area of the SMF are 20 ps/nm/km, 0.06 ps/km/nm2 and 110 μm2, respectively. The dispersion and dispersion slope of the SCF are the same as for the SMF but in the opposite sign and the effective area is 30 μm2. Each SMF has an average loss of 0.2 dB/km at around 1550 nm while the SCF’s loss is 0.24 dB/km, thus the total loss in the loop span is 8.8 dB. The EDFA is set to 8.8 dB gain to compensate for the entire loop loss, assuming flat gain across the whole spectrum. The EDFA noise Figure is 5 dB. Optical band-pass filters with ideal Gaussian curve of 0.048 THz bandwidth are used at transmitter and receiver for fine filtration of the unwanted components and to allow little guard-bands between the neighboring channels. The received 40 Gbit/s signals are optically time division demultiplexed back into 25
10 Gbit/s signals via two DEMUX stages using two clock recovery circuits as seen in the setup diagram. For evaluation, the simulator is set such that it produces performance results every 1 km transmission.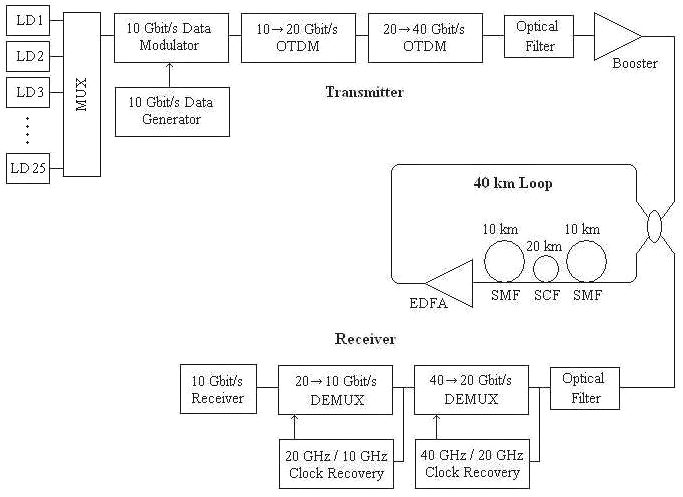 | Figure 1. Experimental setup |
3. Results and Analysis
The performance of the system described above is studied by examining different peak power and pulse-width (through full wave half maximum, FWHM) values of the propagating 25
40 Gbit/s signals against transmission distance. Usually, the performance is evaluated via BER or Q-value of the received signals where good transmission should satisfy BER ≤ 10-9 or Q ≥ 6. This implies that the optimum distance is effectively the maximum distance that meets the above condition. The peak power values used in our test extend between -2 dBm and +2 dBm while FWHM are between 3–5 ps. This is because the performance degrades considerably when using values outside these intervals. In fact, this can be understood as if the peak power is too small, the system would be impaired by noise thus optical signal to noise ratio (OSNR) decreases over short distance. In contrast, if the peak power is too high, the system would be impaired by nonlinearities thus the signal distorts shortly, resulting in high BER. On the other hand, if the pulse-width is too small, the data signal would loose some of its information and thus errors will be observed within short transmission. In contrast, if the pulse-width is too wide, an inter-symbol interference (ISI) between the neighboring bits would appear due to polarization mode dispersion (PMD), resulting in considerable distortion on data over short distance. Based on this, it is necessary to find the optimum values of peak power and FWHM at which signals can reach the maximum possible propagation distance. For accuracy, the simulation is run four times for each test and the results are based on average values. This is required as amplifier produces random noise thus the results slightly deviate every time we run the same test. The first set of results is shown in Figure 2 which presents the maximum transmission distance obtained with Q ≥ 6 versus peak power for selected five wavelengths using different FWHM values. Note that the wavelengths selected are distributed across the entire spectrum to cover as wide range as possible of the wavelengths. It is clear from this set of graphs that the maximum propagation distance differs from one channel to another, and also differs for different parameters. Obviously, the side signals (Ch.1 and Ch.25) often performs better than the mid channels since they have only one side interaction thus less inter-channel crosstalk caused by cross phase modulation (XPM) and four wave mixing (FWM). To determine the optimum parameters, the signals performance is compared for different parameters and the decision is made according to the worst signal behaviors. In more details, for FWHM = 3 ps as shown in (a), good overall performance is achieved for peak power around 1.25 mW (or 1 dBm) where the maximum distance of the worst signal (Ch.13) is 460 km although Ch.1 reached 535 km. Comparing this with the other pulse-width results shown in (b) and (c), good performance is achieved at around 1 mW (0 dBm) peak power for both 4 and 5 ps FWHM. This difference in optimum peak power is understood as for 3 ps, the signal lost little part of its power thus it needed more power to hit nonlinearities. Apparently, the worst case for 4 and 5 ps is also corresponding to Ch.13 that reached 571 km and 476 km, respectively. As a result, the optimum peak power and pulse-width for our system can be 1 mW and 4 ps, respectively. This is because the worst signal (Ch.13) has been able to reach 571 km using these particular parameters while this distance decreases significantly when using other values. Theoretically, a good compromise between noise and nonlinear impairments has been accomplished using the optimum power specified. For pulse-width, the optimum value ensures that the pulses are broad enough to contain full information of the data bits while do not overlap due to PMD effect. However, it is still impossible at this stage to determine the maximum transmission distance for our system which must be based on the worst signal performance among the entire 25 signals using the optimum parameters. To be able to do so, the maximum transmission distance with Q ≥ 6 versus peak power is plotted for all twenty five wavelengths using 4 ps FWHM as shown in Figure 3. From this Figure, it is confirmed that 1 mW peak power is the optimal value for all signals and thus the maximum propagation distance of our system can be 560 km. Again, this distance is effectively the maximum distance reached by the worst two signals Ch.10 and Ch.14 whose wavelengths are 1549.2 and 1550.8, respectively. 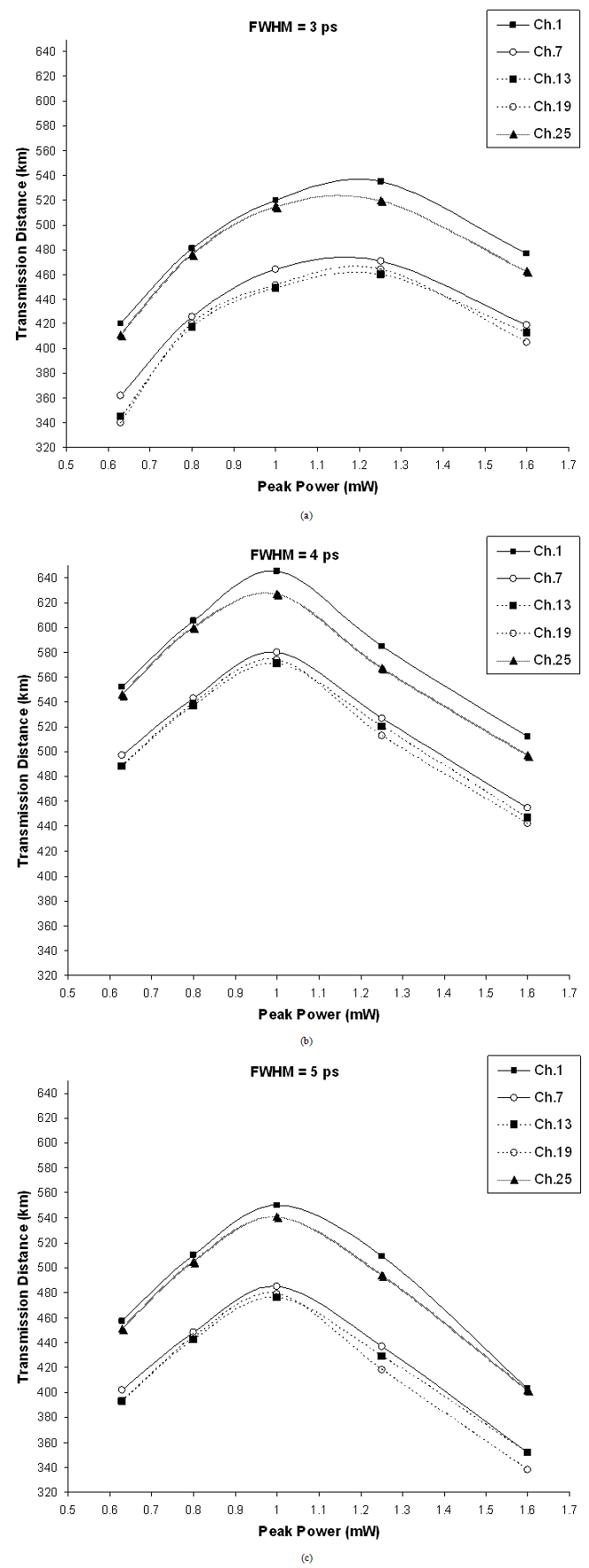 | Figure 2. Transmission distance versus peak power for different values of FWHM: (a) 3 ps; (b) 4 ps; and (c) 5 ps. Channels’ definitions: Ch.1 = 1545.6 nm; Ch.7 = 1548 nm; Ch.13 = 1550.4 nm; Ch.19 = 1552.8 nm; Ch.25 = 1555.2) |
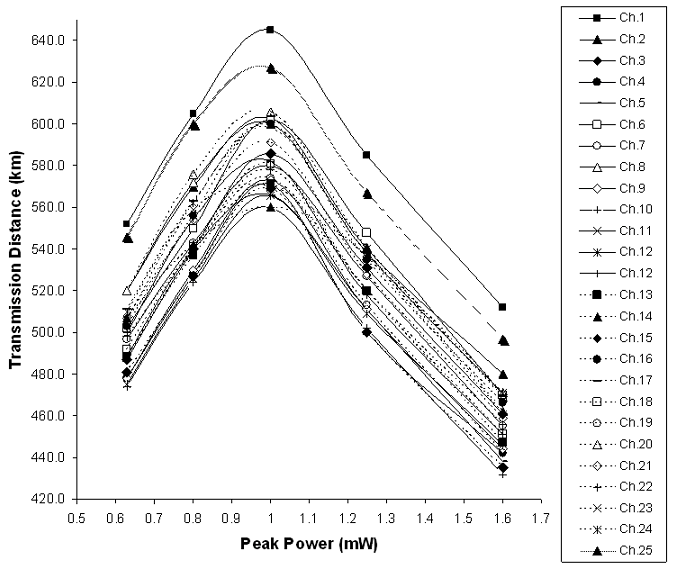 | Figure 3. Transmission distance versus peak power for FWHM = 4 ps for 25 signals. Ch.1 to Ch.25 wavelengths are 1545.6 nm to 1555.2 nm, 0.4 nm spacing (50 GHz) |
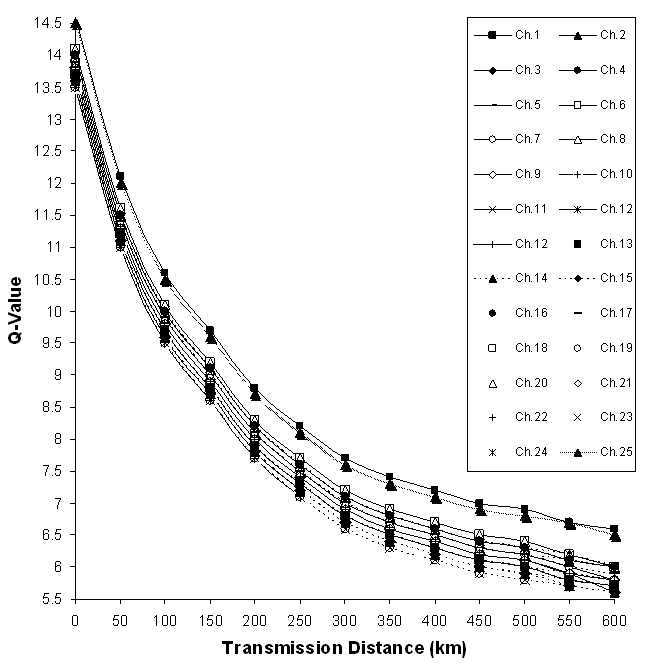 | Figure 4. Q-value versus propagation distance for all channels using peak power = 1 mW and FWHM = 4 ps. Ch.1 to Ch.25 wavelengths are 1545.6 nm to 1555.2 nm, 0.4 nm spacing |
Since the transmission results shown in Figure 3 are based on Q-value measurements, it is essential at this point to show Q-value evolution with transmission distance at the optimum parameters. Figure 4 shows Q-value versus distance for all channels using 1 mW peak power and 4 ps pulsewidth.
4. Conclusions
In this paper, we demonstrated simulation results for 50 GHz spaced 25
40 Gbit/s WDM signals transmission using band-limited RZ modulation format over SMF-Based Large Effective Area Fiber (LEAF). Transmission performance with BER ≤ 10-9 was successfully achieved over 560 km using 1 mW peak power and 4 ps pulse-width for each data signal carrying 231-1 PRBS. The experiment used optical filters with sharp transmission characteristics in both transmitter and receiver.
References
[1] | Jin-Xing Cai, C. R. Davidson, M. Nissov, Li Haifeng, W. T. Anderson, Yi Cai, Li Liu, A. N. Pilipetskii, D. G. Foursa, W. W. Patterson, P. C. Corbett, A. J. Lucero, and N. S. Bergano, “Transmission of 40-Gb/s WDM signals over transoceanic distance using conventional NZ-DSF with receiver dispersion slope compensation”, Journal of Lightwave Technology, vol. 24, No. 1, pp. 191-200, 2006 |
[2] | Sang Soo Lee, Hyunwoo Cho, Sang-Kyu Lim, Kyeong-Mo Yoon, Yong-Gi Lee, and Jesoo Ko, “Field trial of 1.6 Tb/s (40 channels 40 Gb/s) NRZ signals over 511 km standard single mode fiber using conventional optical amplifiers”, IEEE International Conference on Communications (IEEE ICC 2005), vol. 3, pp. 1598-1602, 2005 |
[3] | C. M. Weinert, R. Ludwig, W. Pieper, H. G. Weber, D. Breuer, K. Petermann, and F. K¨uppers, “40 Gb/s and 4 40 Gb/s TDM/WDM standard fiber transmission”. Journal of Lightwave Technology, vol. 17, No. 11, 1999 |
[4] | K. Suzuki, H. Kubota, A. Sahara, and M. Nakazawa, “40 Gbit/s Single Channel Optical Soliton Transmission Over 70000 km using Inline Modulation and Optical Filtering”. Electronic Letters, Vol. 34, No. 1, pp. 98-99, 1998 |
[5] | Antoine Tan, and Erwan Pincemin, “Performance Comparison of Duobinary Formats for 40-Gb/s and Mixed 10/40-Gb/s Long-Haul WDM Transmission on SSMF and LEAF Fibers”. Journal of Lightwave Technology, vol. 27, No. 4, 2009 |
[6] | R. Ludwig, U. Fieste, E. Dietrich, H. G. Weber, D. Breuer, M. Martin, and F. Kuppers, “Experimental comparison of 40Gbit/s RZ and NRZ transmission over standard singlemode fiber”. Electronics Letters, vol 35, pp. 2216-2218, 1999 |
[7] | M. M. Nahas, “40 Gbit/s OTDM Signal Transmission over 1100 km using SMF-Based Effective Area Enlarged Positive Dispersion Fiber”, Canadian Journal on Electrical and Electronics Engineering, Vol. 2, No. 8, pp. 368-370, 2011 |
[8] | M. M. Nahas, “50 GHz Spaced 4 40 Gbit/s WDM Transmission over 700 km Using 6 ps Bandlimited RZ Signals”, Optics and Photonics Journal, vol. 1, pp.137-141, 2011 |
[9] | K. Tanaka, I. Morita, and N. Edagawa, “50 GHz spaced 40 Gbit/s 25WDM transmission over 480 km using bandlimited RZ signals”, Electronics Letters, vol. 37, pp. 775-776, 20 |