Cuitiño Guadalupe1, Esteves Alfredo1, Barea Gustavo1, Marín Laura2, Bertini Renato3
1Environment, Habitat and Energy Institute Mendoza - CONICET, Av. Ruiz Leal s/n, Argentina
2Independent Architect, Mendoza, Argentina
3Green Village Tudunqueral Srl, Mendoza, Argentina
Correspondence to: Cuitiño Guadalupe, Environment, Habitat and Energy Institute Mendoza - CONICET, Av. Ruiz Leal s/n, Argentina.
Email: |  |
Copyright © 2018 Scientific & Academic Publishing. All Rights Reserved.
This work is licensed under the Creative Commons Attribution International License (CC BY).
http://creativecommons.org/licenses/by/4.0/

Abstract
Increasingly, families are choosing to build their homes using earth-based technologies. This is the case in Tudunqueral Ecovilla (eco village) located in Uspallata Valley, Mendoza, Argentina. In this Andes Mountain Area, the houses primarily have been built with “Quincha” (also known as “wattle and daub”). Specifically, this paper aims to evaluate the thermal performance of the eco village’s Multi-Purpose Centre (MPC) which is a “Quincha” construction. Indoor temperature and relative humidity measurements and all external variables of climate (temperature, relative humidity, solar radiation and wind speed and direction) has been registered for winter and summer seasons. Thermography to evaluate local thermal situations of walls, ceilings and floors has been used. An interesting feature is that MPC has a Trombe Wall as passive solar system for heating it. Implementing energy conservation strategies coupled with the use of “quincha” as constructive technology allow for excellent results in the face of the rigorous climate of the mountain environment. It has proven that although low outdoor temperatures of -6°C were recorded, at the same time indoor temperatures was near 10°C, that means a temperature difference (in-out) of around 16°C. As well, while outdoor thermal amplitude reached 26°C, with the optimization of the MPC the thermal range indoors was 6.25°C. And the monetary cost for the optimization is US$3200 which is acceptable for the thermal benefits.
Keywords:
Bio construction, Quincha, Thermal performance
Cite this paper: Cuitiño Guadalupe, Esteves Alfredo, Barea Gustavo, Marín Laura, Bertini Renato, Thermal Performance of “Quincha” Constructive Technology in a Mountainous Region, International Journal of Construction Engineering and Management , Vol. 7 No. 2, 2018, pp. 53-64. doi: 10.5923/j.ijcem.20180702.01.
1. Introduction
The concept of bio construction refers as much to the biology of the construction as it does to an analysis of the relationship between human beings and the built environment, in a quest for a harmonious balance between nature and housing. In the design stage, ecology, building materials and energy should be taken into consideration in order to close the materials loop, i.e., that building waste as well as the structure itself at the end of its useful life, can be recycled into a new construction or returned to nature without producing contaminating waste. In vernacular architecture earth is one of the most important building materials because of its great abundance in all regions of the world, leading many developing countries to use it in the form of different natural construction techniques such as adobe, cob, tapial, and quincha (also known as “wattle and daub”), among others, to build their dwellings. According to Houben and Guillard, 50% of the population in developing countries where at least 20% inhabit urban or marginal urban areas live in earthen dwellings (Houben y Guillard 1994).The technological advances in construction over the last century have meant the relegation of buildings that use natural materials. However in many parts of the world natural architectural resources are used in building as is the case in two of the most populous countries, India and China (Heathcote, 2011). One of the most important properties of an earthen dwelling is its thermal performance due to the fact that the heat inertia of earthen walls helps to maintain interior thermal comfort levels without the need for mechanical heating or cooling, with a subsequent saving in energy consumption (Cuitiño et al, 2010; Esteves et al, 2017). Normally, thermal efficiency is relegated when it comes to building as other matters such as financial costs and the socio cultural context take precedence. A building’s thermal performance does however directly influence the people living in it, therefore it is important that habitability conditions for indoor spaces should translate into comfort in the house and a better quality of life. According with Cunha (Cunha et al; 2014), the tabique is a variant of earth wall that used mixed technic, similar the quincha wall. In his research with tabiques with thermal improvements it used a tabique wall coated with metal corrugated sheets and extrude polystyrene, obtained as a result a reduction of thermal transmittance coefficient of 61%, respect the tabique wall without improvement, of 0.56 W/m2°C.In the case developed by Briga Sá (Briga Sá et al, 2016), worked with tabique wall coated with schist tilesand with extrude polystyrene. In the thermal study allows to verify whether the temperature diagram has the right slopes and leads to a 71% reduction of the thermal transmission coefficient respect the one without insulation, giving a value of 0.46 W/m2°C.Hegediš (Hegediš et al, 2017), had research about rammed earth walls, and obtained that have a good heat capacity, because their homogeneous mass provides their slow heating and cooling. In most cases eliminate the need for cooling and heating of the inner space. The thermal transmittance obtained for rammed walls was 0.351 W/m2K.Shea (Shea et al, 2010), shows that lime-hemp can be used as a replacement for wattle and daub, and obtained that the conductivity of lime-hemp was 0.08 W/m2K for a density of 310 kg/m3 with an inner room variation of 0.9°C respect to the 6.5°C in the exterior temperature.In the research carried out by Whitman (Whitman; 2014) (Whitman et al; 2016), was obtained that hygrothermal properties of adobe construction depend on local soil condition and that the proportion in the mix was not standardized, so can be difficult to fix a thermal conductivity coefficient. However, studies show that there exists a strong correlation between the thermal conductivity of earth as a function of its density and was obtained a thermal value of 0.64-0.79 W/mK with a 1580 kg/m3 density. Also, in the monitoring of a timbur-framed building in situ where the 18% are of wattle and daub walls, the result was a thermal transmittance of 3.25 w/m2K which is equivalent to a concrete wall of 25cm thick.Osvaldo Chiappero states in his book Arquitectura en Tierra Cruda (Raw Earth Architecture) “Raw earth architecture is a technological and cultural manifestation that identifies us with our natural environment and historical development; within it, satisfying the need for shelter in human beings goes hand in hand with the technological responses relating to place. Adobe, tapia, wattle and daub, are extraordinary heat regulators, offering protection from the sun and heat during the day and releasing the accumulated warmth in the cool of the night. These are advantages that were recognised and appreciated by the inhabitants of our region several generations ago” (Chiappero et al, 2003).Nowadays the people who build their houses demand more and more efficient buildings economically and energetically, paying attention to the interior climate within the range of comfort (Minke, 2013).On the other hand, it is undoubtedly that the consumption of fossil energy is driving us towards global change, and of continuing in this rate of consumption, we will have to design and work to live with the consequences that derive from it droughts, floods, increased sea level and excessive pollution (IPCC, 2007). It is necessary to integrate formal and informal knowledge, varied disciplines and all social actors (Penque, 2012).The lifestyle also has a clear impact. In a situation of prosperity, there is greater consumption. With this, it increases the use of resources, the generation of waste and finally the production of more CO2 (Edwards, 2006).It is necessary to have technologies compatible with the environment, using renewable resources, social and ecological values, tending to build buildings of zero energy, which reduce or eliminate dependence with fossil fuels and thus the environmental impact of the building (Guzowski, 2010).To avoid the adverse impacts of such climate change on water resources, ecosystems, food security, health and other harmful effects, it was decided to limit the increase in the overall average temperature to no more than 2°C above the values of the preindustrial era, which implies stabilizing emissions in the range from 445 to 490 ppm CO2 EQ in the atmosphere (IPCC, 2014).Newly developed earth construction techniques demonstrate the value of soil not only for self-construction but also for industrialized construction (Minke, 2013).Despite the prejudices surrounding natural architecture with regard to its structural resistance, heat performance, durability etc, there are a growing number of people who opt for this system to build. This is the case for a group of people that have chosen to build their houses 110 kms from the city of Mendoza in the department of Las Heras in Uspallata Valley. Here an enterprise called Tudunqueral Ecovilla has been launched, an eco village which includes leisure facilities and organic farm plots and whose ideal dwelling’s prime building material is earth. Planned around internal rules and procedures, houses must conform to a building code that respects the environment and utilizes natural resources, with a preference for those materials with certain thermal properties such as wood, stone, earth and cane. “Quincha” is a mixed construction system using cane to form a framework which is then filled with earth, frequently used in the bio construction of earthquake zones owing to its resistance, low weight and flexibility (Cuitiño et al, 2014).To harness and integrate the renewable natural resources of the environment, is coherent, respectful and friendly with the planet and with humanity (Martínez Martínez, 2015). Using renewable energies in combination with an energy-efficient and eco-friendly envelope is an interesting possibility on the road to reducing the housing deficit in any country.The aim of this paper is to evaluate the thermal response of the quincha-built MPC in Tunduqueral Ecovilla in the Andean mountain region. Readings were made in situ over a period of days, the simulation was adjusted using Energy Plus calculation engine and based on the results improvements were subsequently made in order to optimize the building’s thermal and energy performance.
2. Description of the Site
2.1. Geographic Location and Climate
The geographical coordinates of Uspallata, in the department of Las Heras, Mendoza, are the following: latitude 32°5’ S; longitude 69°33’ W and altitude 1891 m.a.s.l. It is located 110 kms from the city of Mendoza, capital of the province of the same name. According to IRAM 11.603 (Spanish acronym for the Argentine Normalization and Certification Institute) [14] it belongs to bioclimatic zone V with cold climate characteristics. The annual requirement for heating in degree days (for base temperature of 18°C) is 2648°C day/year [15]. Figure 1 shows the absolute maximum monthly temperatures (TMXA), the mean temperatures (TM) and the absolute minimums (TMNA), July being the coldest month with absolute minimum temperatures reaching -15°C and January the hottest with an absolute maximum temperature of 36.4°C. Correspondingly, the average daily value of global irradiation on a horizontal surface (GHI) in the month of July is 8.6 [MJ/m2.day] and in January 25.7 [MJ/m2.day]. In addition, Figure 2 shows that in July and September winds are predominantly from the southwest and reach maximum speeds of 20 km/h whereas in January and March winds blow from the southeast with maximum speeds of 15 km/h. Lastly, Figure 3 shows the bioclimatic diagram for the Uspallata Valley demonstrates that the necessary strategies to provide thermal comfort in winter must include passive solar systems (PSS), as well as maintaining low thermal mass and internal gains; in summer, night cooling with natural ventilation should be used to achieve optimum thermal comfort. 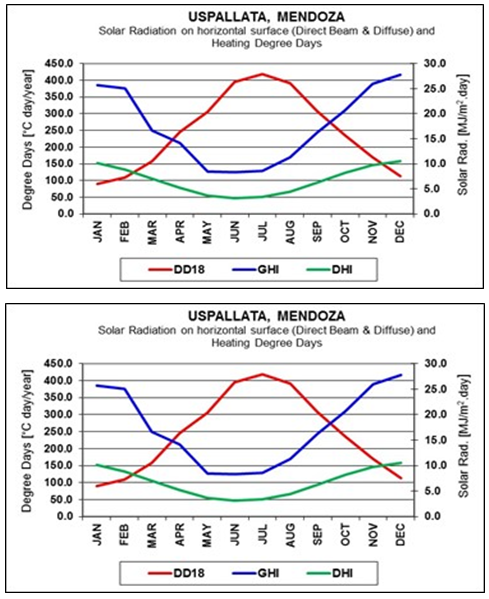 | Figure 1. Mean and absolute temperatures and Solar radiation on a horizontal surface for Uspallata Valley |
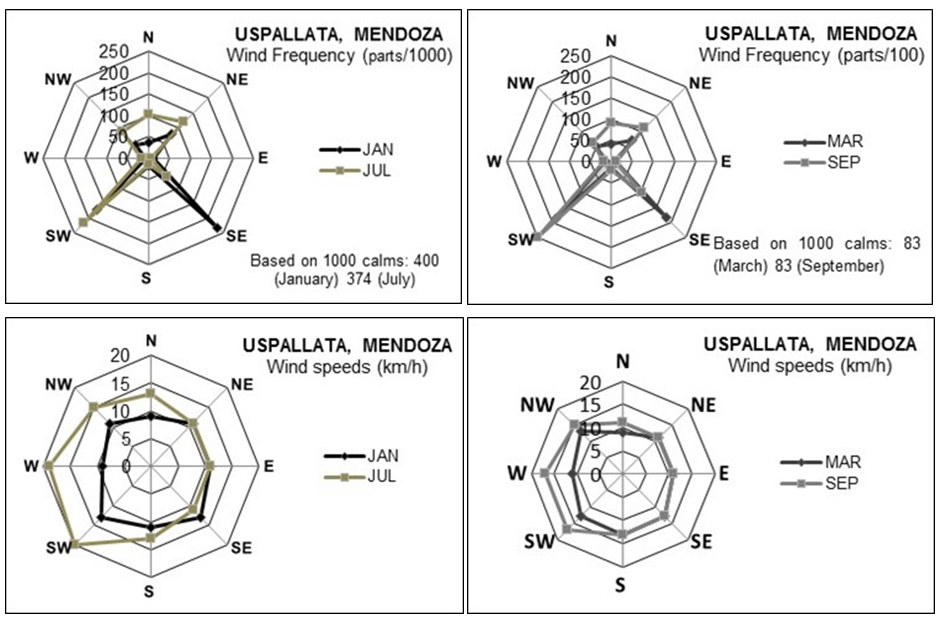 | Figure 2. Wind frequency and speed for Uspallata Valley |
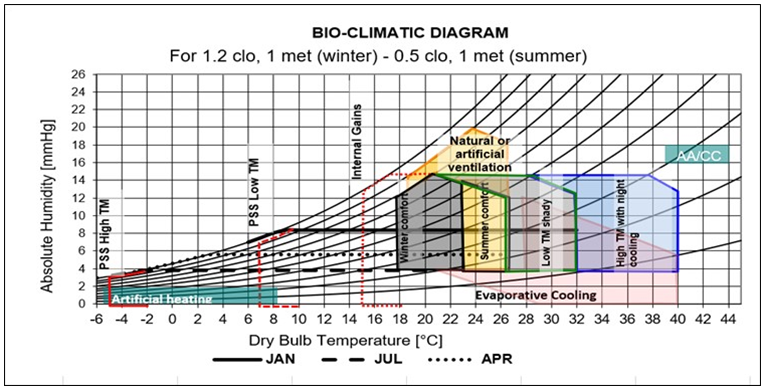 | Figure 3. Bio-climatic diagram for Uspallata Valley |
Table 1 shows monthly temperature variations in Uspallata Valley according to the time of day. From April to October between 12 noon and 6 p.m. the temperature ranges from 10°C to 20°C and between 8 p.m. and 8 a.m. it ranges from 0°C to 10°C. Between November and March the variation is from 20°C to 30°C.Table 1. Hourly and monthly temperature variations in Uspallata Valley 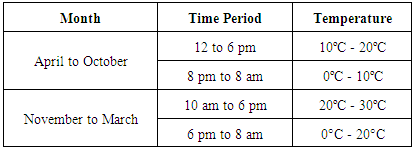 |
| |
|
3. Description of the Multi Purpose Center
The MPC at the Tudunqueral complex is 220.32 square metres in size and comprises the following: a 53.57 m2 multi-purpose room (MPR), a 14.05 m2 kitchen, and 18 m2 for bathrooms and storerooms (see Figure 4). It has a hip roof and windows that face east and west with spectacular views. On the north side it has a Trombe-Michel thermal storage wall with vents that allow convection heating. Figure 5 shows firstly a view of the southeast of the MPC with the main kitchen entrance leading to the MPR; secondly a view of the northwest of the MPC showing the storage wall where 110mm diameter pipes were installed serving as air vents, Figure 6. The last image, Figure 7, shows a view looking northeast from inside the MPR.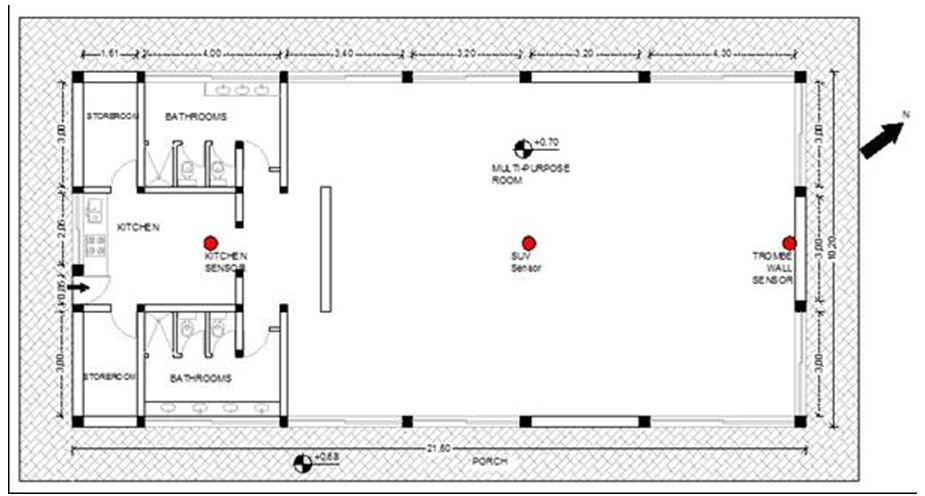 | Figure 4. Floor plan of “Quincha”-built Multi-Purpose Centre |
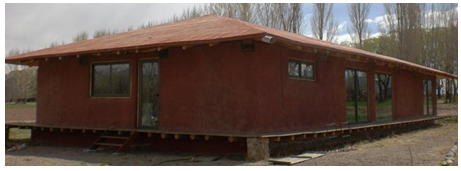 | Figure 5. Southeastern side view of the Multi-Purpose Centre |
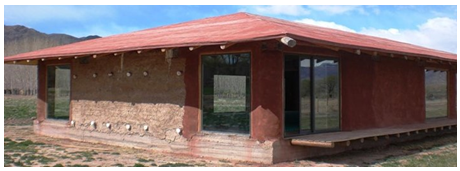 | Figure 6. Northwestern side view of the Multi-Purpose Centre |
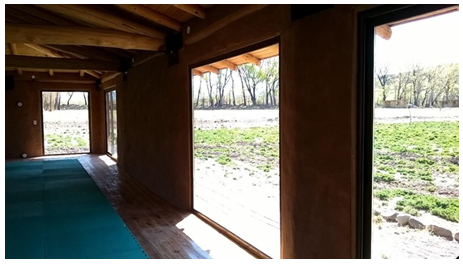 | Figure 7. Inside facing northeast view of the Multi-Purpose Centre |
3.1. Load-Bearing Structure
The project called for the MPC’s foundations to be made of 0.40 m x 0.70m cyclopean concrete, (Figure 8a), with a concrete base underneath each column and a foundation beam or tie-beam whose dimensions are calculated, which ties together all the columns above ground level. This beam allows the interior floor to be raised 0.20m above ground floor level, thus creating an air chamber between the ground and the level of the finished floor known as a floating floor system. Figure 8b shows how the columns look once they are tied together and also shows how the interior floor sits on logs placed on top of the foundation beam and how the floor level is raised with regards to the ground floor level.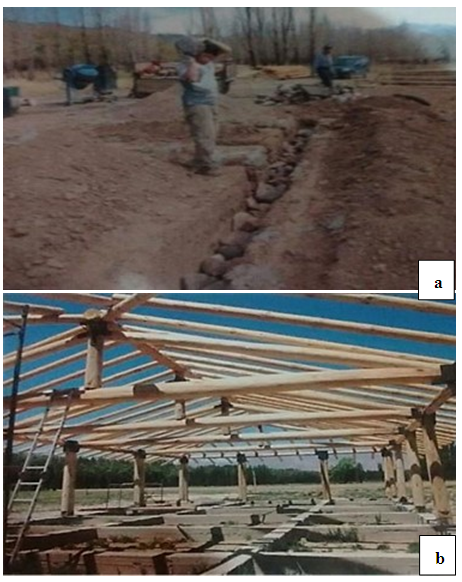 | Figure 8. Multi-Purpose Centre construction details. a- excavating the foundations b- tie-beams and elevation of interior floor |
The load-bearing structure is made up of Paraná pine logs and Eucalyptus logs between 0.8m and 0.20m in diameter (Figure 9a and 9b). Before mounting, the columns are painted with asphalt paint on the end that will come into contact with concrete in order to avoid moisture damage. On blind walls the structure was reinforced by means of wooden diagonal bracing, known as St. Andrew’s cross.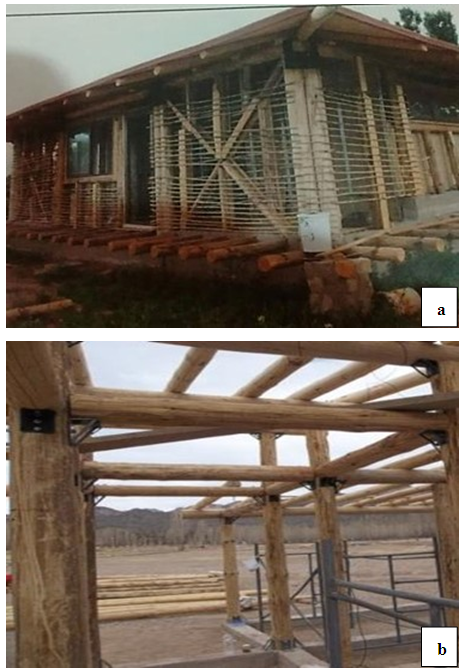 | Figure 9. a- St. Andrew’s cross on walls with cane struts b- Main structure with columns and wooden beams |
Columns and tie-beams are joined using metallic plates, Fischer wall plugs and threaded rods, while columns and wooden beams are joined using dowels with threaded rods and bolts with hexagonal nuts (Figure 10a and 10b). 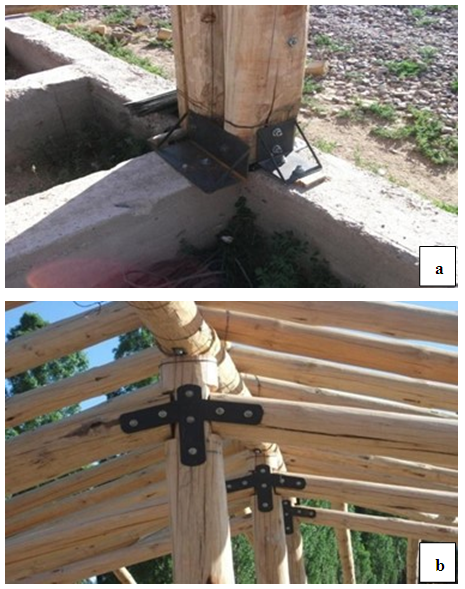 | Figure 10. a- Metal plate connecting timber joints at floor level. b- Metal plate connecting timber joints at ceiling level |
3.2. Thermal Characteristics of the Building Envelope
All the opaque walls are made using the “Quincha” technique whereby cane or wooden slats are placed horizontally and/or diagonally forming a framework on the walls; the spaces in between the framework are then filled in on either side with a mixture of clay, sand and straw, until the wall is 0.30m thick (Figure 5a). The glazing elements in the enclosing walls are made up of aluminium casings for doors and windows and all window panes are hermetic double glazed (HDG). The roofing materials consist of two layers of tongue and groove, waterproof insulation with a Tyvek-like membrane, heat insulation with 50mm fibreglass wool batts and a coloured geotextile finish. The eaves are 0.60m wide to protect the “Quincha” walls from the rain (Figure 11).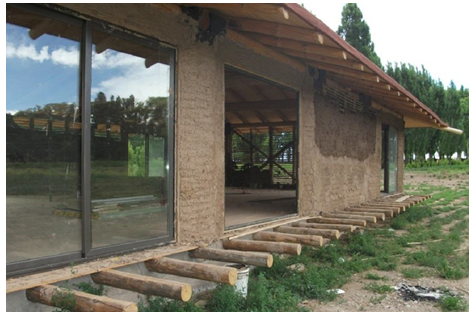 | Figure 11. 60cm-wide eaves protect the “Quincha” walls from the rain |
Table 2 shows the thermal conductivity, density and specific heat of the “Quincha” walls, windows and roofing; the insulating properties of the walls were obtained from prior research data [16] and those of the windows and roofing from the IRAM 11.601/02 Standard [17].Table 2. Thermal properties of the MPC’s constructive systems 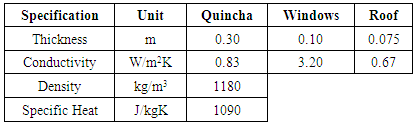 |
| |
|
3.3. Passive Solar System
The north-facing wall (Equator facade) was designed as a passive solar system of Tombe-Michel storage wall. It is also made of “Quincha” of 0,30m thickness and with 110mm diameter PVC pipes that allow hot air to enter on the upper level and cold air to exit on the lower level (Figure 12). In the photograph below the external glazing has yet to be installed. 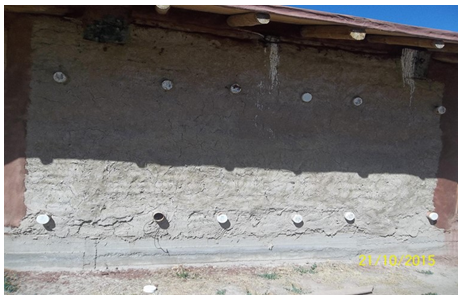 | Figure 12. The Trombe-Michel wall without its external glazing and with PVC pipes as air vents |
4. Thermal Situation of Multi-Purpose Centre
The thermal situation of the MPC was determined firstly by measuring the representative variables: indoor temperatures and relative humidity, outdoor temperatures and relative humidity, solar radiation on a horizontal surface and winds (speed and direction). Secondly, thermal simulation was carried out in order to adjust the parameters and lastly a simulation based on other times of the year was undertaken, thus evaluating the MPC’s thermal response.
4.1. Measuring Representative Variables
Hobo U12-001 Data Loggers were used for measuring the representative parameters such as temperature and relative indoor humidity. These were placed in strategic areas (see Figure 4); a) in the kitchen b) in the center of MPR c) on the storage wall: for the latter measurements a Hobo U12 Data Logger for a Type T thermocouple was introduced from the outside into the lower part of the “Quincha” wall.In order to measure outdoor conditions such as temperature, relative humidity, solar radiation and wind speed, a Pegasus-brand weather station was provided. The length of the measuring period was one week and it was carried out in springtime, between the 24th and the 30th of September, with a recording interval of 15 minutes. In addition, thermographic photos were taken with an IR Fluke Ti55 camera which has a spectrum band of 8 µm to 14 µm, a thermal sensitivity of ≤ 0.05°C and measures a temperature range of -20°C to 600°C. The camera enabled thermal images to be taken which revealed the thermal behaviour of the “Quincha” walls. In these images every pixel contains a specific temperature value for which the emissivity settings were adjusted according to the type of material measured. To analyze the images, SmartView 2.1.0.10 software which assigns a specific colour to each temperature value, was used. To calculate emissivity (ε) a Type T thermocouple sensor connected to a Hobo U12 Data Logger was used and the value of the “Quincha” wall’s surface temperature was compared with data registered by the thermal image in such a way that the material’s emissivity value made it possible to match the thermocouple temperature reading with that of the thermography [18].
4.2. Analysis of the Recorded Data
Figure 13, shows temperatures recorded during the study period in the MPR and kitchen, outdoor temperatures and solar radiation on a horizontal surface. It shows that for the weekly period the first three days were partially cloudy followed by four clear days in which solar radiation on a horizontal surface at solar noon reached 860 W/m2.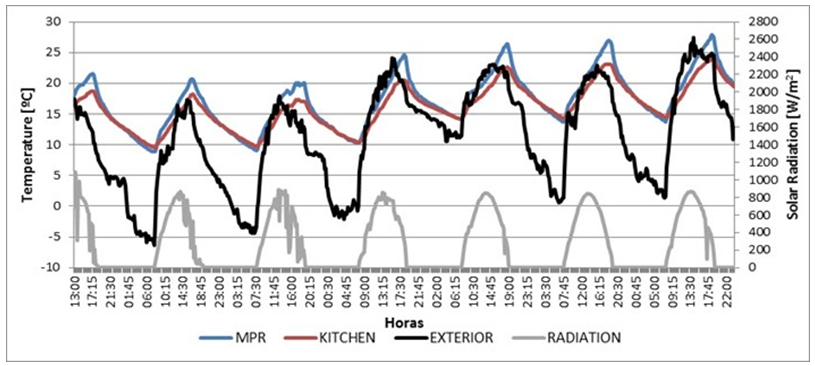 | Figure 13. Comparative temperature curves for the MPR, kitchen, exterior and solar radiation |
The results are typical of valley climates that are characterized by high thermal amplitude. In fact maximum outdoor temperatures fluctuated between 17°C and 27°C and minimum temperatures between 9°C and -6°C, representing a temperature range of between 13°C and 26°C (See Table 3 for daily readings). The first three days there were frosts, while on the fourth day the minimum was considerably warmer as a result of north winds. As of the fifth day the high temperature range became apparent again with very low temperatures recorded in the early morning (close to 0°C).The MPR and the kitchen, subjected to the same climatic conditions and during the same time period, behaved in a similar way. The MPR registered maximum temperatures of between 20°C and 27°C and minimums of between 8°C and 14°C, while the kitchen registered maximums of between 17°C and 24°C and minimums of between 9°C and 14°C, with a temperature range of between 5°C and 10°C. Neither of the rooms had auxiliary heating during the measuring period, but relied exclusively on accumulated solar energy. Results show a difference between the maximum indoor and outdoor temperatures of around 14°C. Because of the thermal inertia of the “Quincha” walls, the indoor temperature drops slowly during the night, allowing the temperature to recover rapidly during the following day. The difference between the MPR’s maximum temperature and that of the kitchen is due to the former having the Trombe wall which absorbs most of its energy during the afternoon when indoor temperatures peak. However, the minimum temperature value for the two rooms remains the same, indicating that the entire interior of the building cools in the same way.Table 3 details the maximum and minimum values and the temperature range recorded daily during the study period. It shows that the MPR and the kitchen display similar thermal behaviors.Table 3. Temperature data recorded during the week 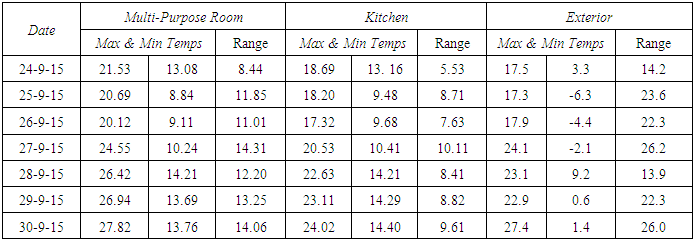 |
| |
|
Temperatures registered on the outside of the Trombe wall (at a depth of 1 cm) as detailed in Figure 14, are between 44°C and 49.7°C, while temperatures registered on the inside of the wall approximate those recorded in the MPR. At night the surface of the Trombe wall cools down and registers intermediate indoor/outdoor temperatures. The graph highlights the importance of passive solar systems in a favorable climate such as this, with its high percentage of sunny days. 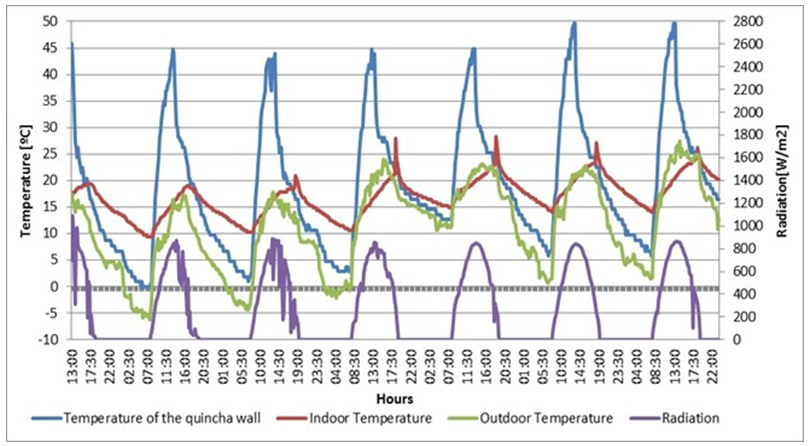 | Figure 14. Temperatures registered for the Trombe wall |
Thermographic images taken of the roof (Figure 15) clearly show the thermal bridges causing heat loss at 11 a.m. when the photo was taken. Surface temperatures were 25.3°C and in areas without thermal bridges the temperature was 17.2°C, indicating a heat loss of approximately 8.1°C. Insulating the area would eliminate all the thermal bridges and reduce thermal variation.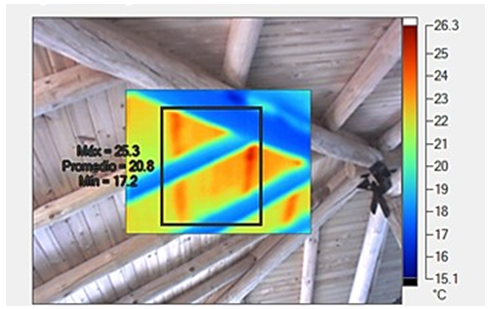 | Figure 15. Thermographic image of the MPC’s roof at 11 am |
5. Thermal Simulation
Thermal simulation was carried out in order to adjust the MPC interior thermal response values during the measuring period. Ultimately it revealed what will occur in the building at other times of the year. Simulation was carried out using the 8.4.0 version of the Energy Plus program, developed by the United States Department of Energy in 2011 to enable building energy and thermal load analysis. It employs a dynamic regime that allows energy systems to interrelate with the characteristics of the building envelope.Geometric representation of the MPC was carried out using the Open Studio Plugin which was designed for compatibility with Google SketchUp and which enables 3D graphic representation (see Figure 16), and differentiation of the geometry of walls, windows, floors, roofs and eaves. In the modeling adjustment phase, climatic data measured in situ was employed and for the optimization phase a climate data file was created based on a design day using meteorological data obtained from the National Meteorological Service. 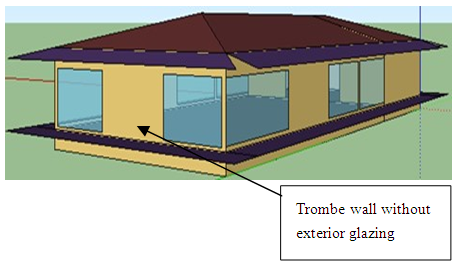 | Figure 16. SketchUp model of the MPC |
The properties of the building materials used in the MPC and the climatic data for the study period (from the 24th to the 30th of September) were uploaded. Thermal and energy simulation was then carried out and the adjustment between the recorded data and the simulated data was made, Figure 17. 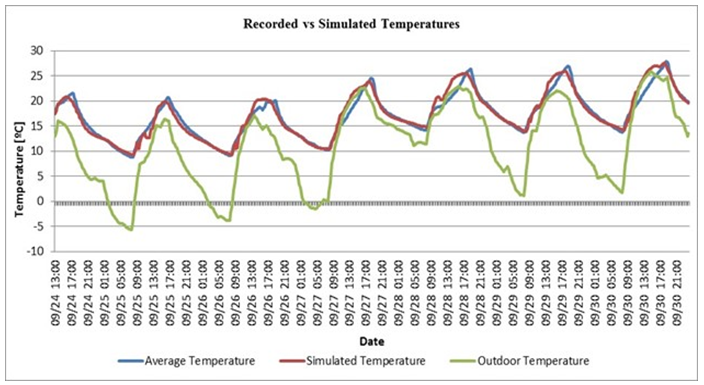 | Figure 17. Recorded and simulated temperatures in the MPC and recorded outdoor temperatures |
Figure 18 highlights the degree of correlation between temperature values recorded in situ and those obtained via simulation where the coefficient of determination is R2 = 0.9482, resulting in a reliable model for further simulation.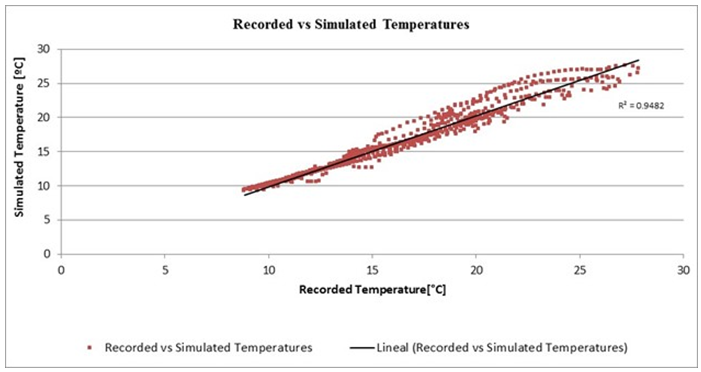 | Figure 18. Correlation between average temperature values vs simulated values |
Simulation of the MPC was then carried out with the Trombe wall exterior glazing in place. The storage wall was built with 0.30 m thick “Quincha”, the side facing the sun was painted with dark, 0.85 heat absorbing paint (black paint) and the external enclosure consisting of double glazing was put in place, as shown in the SketchUp image in Figure 19. 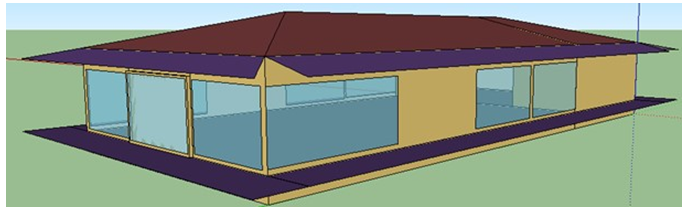 | Figure 19. SketchUp model of the MPC incorporating the storage wall |
Computer simulation of the MPC for the month of July was carried out using data from the National Meteorological Service relating to Uspallata (weather statistics for the 2000-2010 period). The corresponding climate data file was generated. The results can be seen in graphic form (Figure 20). As is apparent, by incorporating the exterior glazing into the Trombe wall the simulation indicates an increase in temperature during daylight hours with a peak of around 2°C close to midday. Once the model was adjusted, the improvements were implemented and the response registered. Performance was tested by means of thermal simulation as the wall’s exterior glazing had yet to be installed.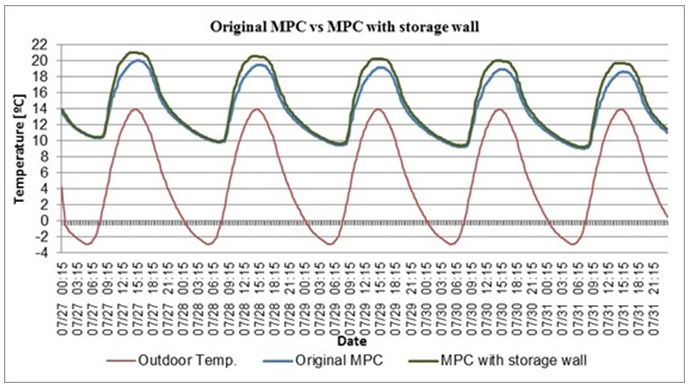 | Figure 20. MPC’s performance with and without the storage wall |
5.1. Optimization of the MPC
Optimization of the MPC’s design was undertaken by reducing the glazed area (high thermal transmittance) whilst increasing the opaque wall surface, thus reducing heat loss. This involved a third stage during which the following was considered: - The glazed surface area was reduced by 50% on the eastern and western facades, (Figure 21) and curtains were drawn all night until 8 a.m.- Weather-stripping was applied on windows and doors- A suspended ceiling of 5cm thick expanded polystyrene was installed to improve heat resistance in the roof.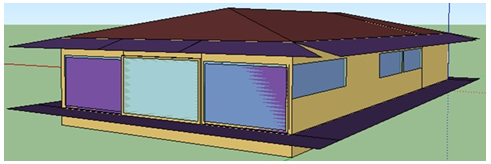 | Figure 21. Google SketchUp rendering showing reduction in glazed area |
Figure 22 shows simulation of the thermal improvements made to the MPC. It is possible to see that the minimum indoor temperatures have increased and the maximum indoor temperatures have remained the same. At night the downwards curve is slower, allowing for rapid temperature recovery during the next day. The thermal range reveals 11.3°C for outdoor temperatures with minimums of 3.3°C and maximums of 14.6°C. In the case of the MPC with the storage wall the results are 11.7°C, but with minimums of 8.4°C and maximums of 20.1°C, higher than those recorded outside. Finally, the MPC’s temperature range with the improvements is 6.25°C, not only lowering the temperature variation between day and night but also raising the minimums to 14.3°C while the maximums remain the same. Moreover, there was no auxiliary heating in the internal space to help maintain thermal comfort. 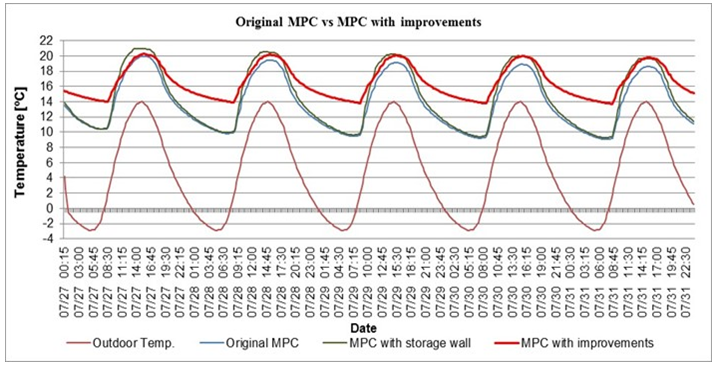 | Figure 22. Simulation of the thermal improvements applied to the MPC vs the original MPC |
At the same time, the MPC’s performance was simulated for the month of January, in summertime (southern hemisphere), as see in Figure 23. As in the previous simulation a design day was employed based on data from the National Meteorological Service. The results were stable with little variation in temperature, while outside the temperature variation was 18°C and inside 8.1°C, thus validating evidence that the thermal improvements proposed for winter are beneficial in summer and that night ventilation for cooling is to be recommended.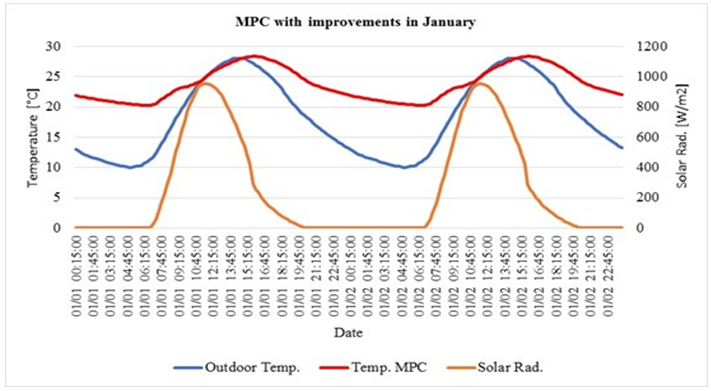 | Figure 23. Simulation of MPC with thermal improvement for design day of January |
Lastly, considering the optimization of the MPC, which include the items described at the start of point 5.1, the investment cost is US$ 3200, this cost includes materials and labour. Although the cost coud be considered high, the thermal benefits would be possitive and the inner temperature will remains stable in time during summer and winter, which is desirable for the people confort.
6. Conclusions
In this paper has been studied the performance of a building that have “Quincha” technology and are situated in a mountainous region such as the Uspallata Valley in Mendoza Province, Argentina. It has proven that although low outdoor temperatures of -6°C were recorded, at the same time indoor temperatures was near 10°C, that means a temperature difference (in-out) of around 16°C. Likewise it was observed that thanks to the thermal inertia inherent to earthen walls and to the double glazed windows, indoor temperature dropped slowly, allowing for a rapid recovery during sunlight hours. It is important to note that while outdoor thermal amplitude reached 26°C, indoors it was 14°C at the most. Energy Plus simulation was able to confirm that with a reduction in the area of window glass and with expanded polystyrene in the ceiling, which have an investment cost of US$ 3200, including materials and labour, the thermal range indoors was significantly reduced, down to 6.25°C. Subsequently in summer a favorable response to the improvements was recorded, with an indoor temperature range of 8.1°C as compared to 18°C outdoors.
ACKNOWLEDGEMENTS
Special thanks go to the owner of Tudunqueral Ecovilla, Renato Bertini, and to the architect Laura Marín for their goodwill and cooperation in carrying out this research.
References
[1] | Houben, H; Guillaud, H. (1994). Earth construction: a comprehensive guide. Intermediate Technology Publications. |
[2] | Heathcote, K. (2011). The thermal performance of earth buildings. Informes de la Construcción, 63(523): 117-126 doi: 10.3989/ic.10.024. |
[3] | Cuitiño G; Esteves A; Maldonado G, Rotondaro R. (2010). Análisis y reflexiones sobre el comportamiento higrotérmico de construcciones con quincha. Estudio del caso de un taller experimental en Mendoza. Revista ÁREA. 0328-1337. |
[4] | Esteves A. (2017). Arquitectura bioclimática y sustentable. Teoria y práctica de la conservación de la energía, sistemas solares pasivos y enfriamiento natural de edificios. ISBN 978-987-42-5102-2. |
[5] | Cunha, Sandra; Jorge, Pinto; Anabela, Paiva; Briga-Sá, Ana; Nuno, Soares; Humberto, Varum and Débora Ferreira. (2014). A contribution for the improvement in thermal insulation of tabique walls coated with metal corrugated sheets. Buidings Services Engineering. Research & Technology. DOI: 10.1177/0143624414558720. |
[6] | Briga Sá, Ana; Sandra, Pereira; Nuno Soares; Jorge Pinto; Joao, Carlos Lanzinha and Analbela Paiva. (2016). An approach on the thermal behaviour assessment of tabique walls coated with schist tiles: Experimental analysis. Energy and Buidings 117. Pp. 11-19. |
[7] | Hegediš, Ivan; Karaman, Golub; Čeh, Arpad; Đurić Neđo; Kukaras, Danijel and Vunjak Danilo. (2017). Energy sustainability of rammed earth buildings. Archives for Technical Sciences. DOI: 10.7251/afts.2017.0917.039H. Pp. 39-48. |
[8] | Andy, Shea; Mike, Lawrence and Pete, Walker. (2012). Hygrothermal performance of an experimental hemp–lime building. Construction and Building Materials 36. Pp. 270-275. |
[9] | Whitman, Christopher J. (2014). Heritage earth construction and hygrothermal comfort: The challenge of rebuilding in Central Chile. Key Engineering Materials Vol 600. Pp 186-195. doi:10.4028/www.scientific.net/KEM.600.186. |
[10] | Whitman, Christopher J and Oriel Prizeman. (2016). U-value Monitoring of Infill Panels of a Fifteenth-century Dwelling in Herefordshire, UK. Association for Preservation Technology. ISSN: 0044-9466. |
[11] | Chiappero, Rubén y Supisiche, María. (2003). Arquitectura en tierra cruda. Editorial Nobuko. ISBN: 987-20641-5-6. |
[12] | Minke G. (2013). Manual de construcción con tierra: la tierra como material de construcción y su aplicación en la arquitectura actual. 4° Ed. BRC Ediciones. Bariloche. Argentina. |
[13] | IPCC, (2007). Cambio Climático 2007 – Informe de Síntesis. Panel Intergubernamental para el Cambio Climático. OMM – PNUMA. 2007. |
[14] | Penque W. (2012). Los desafíos de la economía verde. 1° Ed. Ediciones Kaicron, Buenos Aires. |
[15] | Edwards B. (2006). Rough guide to sustainability (Spanish edition). Ed. G. Gili. Barcelona. |
[16] | Guzowski M. (2010). Energía Cero. Arquitectura Contemporánea. Estética y Tecnología con Estrategias y dispositivos de ahorro y generación de energía alternativa. Ed. Blume. 1ª Ed. Barcelona. |
[17] | IPCC. (2014). Renewable Energy and Climate Change. Cambridge University Press, Cambridge, United Kingdom and New York, NY, USA. |
[18] | Cuitiño G; Maldonado G; Esteves A. (2014). Analysis of the Mechanical Behavior of Prefabricated Wattle and Daub Walls. International Journal of Architecture, Engineering and Construction. Vol 3, Nº 4. Pp. 235-246. ISSN 1911-1118. |
[19] | Martínez Martínez A. (2015). Bioconstrucción. Ediciones i (Silversalud, S.L.). Madrid. |
[20] | IRAM 11.603. (1996). Acondicionamiento térmico de edificios. Clasificación bioambiental de la República Argentina. Buenos Aires. |
[21] | Esteves, A; Gelardi, D. (2008). Método para el cálculo de grados día de cualquier temperatura base y cualquier localidad. Avances en Energías Renovables y Medio Ambiente Vol. 12. Impreso en la Argentina. ISSN 0329-5184. Pp. 153-158. |
[22] | Cuitiño G., Esteves A., Maldonado G., Rotondaro R.. (2015). Análisis de la transmitancia térmica y resistencia al impacto de los muros de quincha. Informes de la Construcción. Vol. 67, 537, e063. doi: http://dx.doi.org/10.3989/ic.12.082. |
[23] | IRAM 11.601. (2002). Aislamiento térmico de edificios. Métodos de cálculo. Argentina. Buenos Aires. |
[24] | ASTM Standard E 1933-99a. (2006). Standard Test Methods for Measuring and Compensating for Emissivity Using Infrared Imaging Radiometers. |