Jacqueline McComb , Sonya Hentz , Gloria S. Miller, Maria Begonia,
Plant Physiology/Microbiology Laboratory, Department of Biology, P.O. Box 18540, College of Science, Engineering and Technology, Jackson State University, 100 Lynch Street, Jackson, Mississippi, 39217, USA
Correspondence to: Sonya Hentz , Plant Physiology/Microbiology Laboratory, Department of Biology, P.O. Box 18540, College of Science, Engineering and Technology, Jackson State University, 100 Lynch Street, Jackson, Mississippi, 39217, USA.
Email: |  |
Copyright © 2012 Scientific & Academic Publishing. All Rights Reserved.
Abstract
Phytoremediation is the use of living plants for the removal of contaminants from soil, water, sediments, and air. Soil contamination by heavy metals (HMs) is an important environmental concern. The success of phytoextraction, a subset of phytoremediation, as an environment clean-up technology depends on several factors including the extent of soil contamination and the bioavailability of the metal. The aim of phytoextraction research is to identify metal-tolerant plants that are capable of uptake and efficient translocation of HMs to harvestable above-ground organs. The main objective of this study was to assess the metal tolerance of coffeeweed (Sesbania exaltata Raf.) after exposure to lead (Pb) solutions. Sesbania plants were grown for 30 days at the Jackson State University greenhouse prior to exposure to different concentrations (0, 0.1, 1.0, 5.0, 10.0, 20.0 μM Pb) of lead nitrate. Four 30-day old seedlings were grown hydroponically in each designated 15-mL cup containing the Pb treatments. Plants were harvested after various exposure periods (0, 2, 5, 8, 12, 15 days). Morphological characteristics were evaluated, and lead contents were quantified by Inductively Coupled Plasma - Optical Emission Spectrometry (ICP-OES). Phytochelatin synthesis was determined indirectly by quantifying the acid - thiol contents of roots and shoots. Our results indicated that lead tissue uptake increased with increasing concentrations of lead (i.e. 20.0 μM versus 1.0 μM). However, plant roots and shoots displayed symptoms of toxicity as evidenced by their decreased biomass with increasing concentrations of lead. Also, plants exposed to higher Pb concentrations exhibited mild chlorosis. Overall, Sesbania was relatively tolerant to lead. Although acid - soluble thiol contents appeared to correlate with Pb uptake especially at higher concentrations of Pb treatments, phytochelatin synthesis as a metal-tolerance mechanism in Sesbania is inconclusive at this time. Further experiments are warranted to elucidate whether such tolerance mechanism exists in Sesbania.
Keywords:
Lead, Phytoremediation, Sesbania Exaltata
1. Introduction
Since the Industrial Revolution, mankind has been introducing various toxic pollutants into the environment at an alarming rate[1, 2]. These pollutants are a cocktail of various organic compounds and heavy metals (HMs) which can drastically affect human and animal health and ecosystems[3]. Heavy metals such as lead (Pb) are of major concern because of their persistence in the environment[4]. Lead is a toxic pollutant mainly introduced into the environment through smelting processes[5]. Although Pb has no known functions in biological systems, it can impact the central nervous system especially in children leading to reduced growth of the brain[6]. It is generally ranked the number one heavy metal pollutant and number two of all hazardous substances by the Agency for Toxic Substances and Disease Registry[7, 8].Cognizant of their effects on human and animal health and ecosystems, it is imperative to reduce the concentrations of HMs such as Pb from contaminated soil and water sources. Numerous engineering-based technologies have been used to remediate metal-contaminated soils and aquatic systems but they are very expensive. Phytoremediation is becoming a more cost-effective alternative[9] because of the productive interdisciplinary cooperation of plant biochemists, molecular biologists, soil chemists, agronomists, environmental engineers, and federal and state regulators. The metals targeted for phytoremediation include Pb, Cd, Cr, As, and various radionuclides. The harvested plant tissue, rich in accumulated contaminant is easily processed by drying, ashing or composting[10].Phytoextraction involves the recurrent cropping of plants in polluted soil, until the metal concentration is reduced to regulatory levels. The ability of the plants to account for the decrease in soil metal concentrations is a function of the extent of metal uptake and biomass production. Both attributes play an important role in achieving regulatory acceptance. Supposedly, metal exclusion can be determined by metal concentration within the plant, multiplied by the biomass produced[11]. Therefore, a suitable phytoextractive species must be able to tolerate the toxic metal and produce high biomass yield.This study was conducted to 1) evaluate the growth and Pb accumulation by Sesbania exaltata plants exposed to various levels of Pb (supplied as lead nitrate) at different growth periods, 2) determine whether S. exaltata plants can tolerate and translocate high concentrations of lead, and 3) assess phytochelatin (PC) synthesis in S. exaltata.
2. Materials and Methods
2.1. Pre-Metal Treatment
Sesbania exaltata seeds were obtained from Azlin Seed Co., Leland, MS. Appropriate amounts of seeds were soaked overnight in 750 mL of deionized distilled water (ddw). Pre-soaked seeds were germinated in aluminum pans containing moist quartz sand. Germinated seedlings were irrigated regularly with tap water based on the evaporative demand. To ensure that the plants were acclimated, 40 mL of nutrient solution were added as the levels of tap water were replenished. Uniform, 30-day old seedlings (4/cup) were transferred to each designated (20 oz.) polystyrene foam cups containing a standard nutrient solution and covered with respective plastics lids.
2.2. Hydroponic Culture
Thirty day-old seedlings (4/cup) were placed through perforations made on the lids, and each seedling was separated and secured by wrapping a cotton plug at the stem-root junction (i.e., basal end of the hypocotyls), thus, ensuring the immersion of the whole root system.A modified Hoagland’s nutrient solution was added daily to replenish evapotranspiration loss. During the experimental period, all plants were maintained at the Jackson State University naturally lit greenhouse. Plants were harvested at designated times after initial Pb exposure.Six hours after initial Pb exposure, S. exaltata were harvested. This represented the day 0 exposure. To ensure that all excess ()2 were eliminated on the root's external surfaces, the roots were washed with deionized, distilled water then placed in paper napkins in separate plastic containers.Two of the four plants were measured to obtain the shoot lengths and root lengths, then segregated into shoots and roots. The separated roots and shoots were placed in designated paper bags, dried in 80 °C oven for at least 48 hours and weighed to obtain their respective dry biomass. The roots and shoots of the two remaining plants per cup were separated, quickly frozen in liquid nitrogen to prevent oxidation, and immediately placed in designated 25 mL sample tubes. Frozen samples were stored at -80 °C in a Thermo Electron Corporation Forma -80 °C ULT freezer until analyzed for phytochelatin contents.
2.3. Phytochelatin (PC) Analysis
Phytochelatin analysis was carried out according to Keltjens and Beusichen[12]. Plant parts (roots and shoots were homogenized separately for 5 minutes in 10 mL of extraction mixture with a mortar and pestle on ice under nitrogen gas to prevent oxidation. The homogenates were placed in centrifuge tubes and cooled on ice. When twelve tubes filled with Sesbania homogenates were collected, they were centrifuged in a Beckman Optima XL 100K Ultra Centrifuge for 15 minutes at 10,000 revolutions per minute (rpm) at 4°C. The supernatant was then decanted into 15 mL BD falcon tube. Phytochelatin concentrations were determined indirectly by assessing the absorption of total acid-soluble thiols, total glutathione, and oxidized (GSSG) glutathione. The total acid-soluble thiols were determined using Ellman’s reagent[13]. Total glutathione and oxidized glutathione were established using GSSG recycling method[14].
2.4. Acid Digestion
The plant parts were placed in corresponding labeled brown paper bags and dried in a Precision Mechanical Convection Oven at 70 °C for at least 48 hours. Dried samples were weighed with a Mettler AE 260 Delta Range to obtain the biomass. Lead contents from plant tissues were extracted using a modified nitric acid-hydrogen peroxide procedure according to U.S. Environmental Protection Agency[15] EPA SW-846 Method 3040B. Approximately 100-200 mg of dried tissue plant samples were placed in a 250 mL Erlenmeyer flask and 40 mL of 50% nitric acid were added and allowed to set overnight. Samples were placed on a hot plate (high setting) and refluxed for 15 minutes until yellow liquid appeared. The solutions were allowed to cool for 10 minutes and 10 mL of 50% nitric acid (HNO3) were pipetted into each sample. The samples were then refluxed for 30 minutes and then allowed to cool for 10 minutes. The samples were then completely dissolved in 5 mL of concentrated nitric acid. The oxidized solution was allowed to cool and 2 mL of ddw and 3 mL of 30% hydrogen peroxide were added. The digested solution was heated until the effervescence diminished. Another 7 mL of 30% hydrogen peroxide were added in 1 mL intervals and the digestate was heated again and reduced to approximately 5 mL. After heating, the solution was diluted to 20 mL with deionized water and the digestate was filtered through filter paper (Whatman No. 1). The final volume was adjusted to 25 mL with ddw.
2.5. Analysis of Lead Content
Lead contents of each sample were quantified using Inductively Coupled Plasma-Optical Emission Spectrometry (ICP-OES) (Perkin Elmer Optima 3302 DV). A standard curve was established using 6 Pb concentrations (0.1, 1.0, 2.0, 5.0, 10.0, and 15.0 ppm Pb). Lead contents were calculated in mg/kg using the absorbance readings of the digestates.
2.6. Statistical Analyses
The data were analyzed using Statistical Analysis System (SAS Version 9). Data were subjected to a one way ANOVA with replication. Treatment comparisons were done using Fisher’s Least Significant Difference (LSD) test (p < 0.05).
3. Results and Discussion
Shoot biomass generally increased with Pb exposure periods from day 0 to day 15 (Figure 1). Greater biomass increases were more remarkable at 12 days after initial exposure. Also, for each exposure period (e.g., 0, 2, 5, 8 days), there were no significant differences in shoot biomass regardless of Pb treatments. However, there were apparent differences in shoot biomass at 12 and 15 days after initial exposure.Decreases in biomass during these two exposure periods were evident in plants exposed to 1.0 and 5.0 μM Pb. Figure 2 specifically displays the shoot biomass of S.exaltata in response to various concentrations of Pb at 15 days after initial exposure. Shoot biomass was significantly reduced in plants treated with 1.0 and 5 μM Pb. Figure 3 displays an image of day 15 after initial exposure to Pb treatments that corresponds with Figure 2. As shown in Figure 3, S. exaltata plants exposed to 5.0 μM of Pb showed dramatic growth reduction compared to the control. The image also shows that S. exaltata were tolerant to Pb.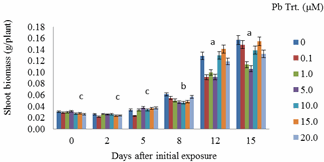 | Figure 1. Shoot biomass (g/plant) of S.exaltata exposed to different Pb concentrations and growth periods. Day means with common letters (on top of bars) do not differ significantly according to LSD test (p < 0.05). Each bar represents the mean + standard error of four replications. |
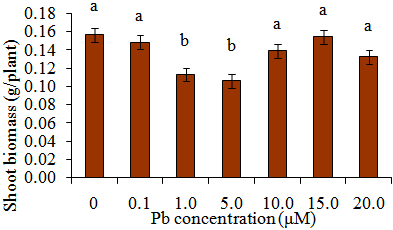 | Figure 2. Shoot biomass (g/plant) of S.exaltata 15 days after initial exposure to Pb. Treatment means with common letters do not differ significantly according to LSD test (p < 0.05). |
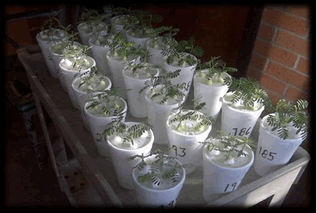 | Figure 3. The morphology of S. exaltata at 15 days after initial exposure to different Pb (μM) treatments. |
Generally, shoot lengths of S. exaltata increased with longer exposure periods to Pb treatments (Figure 4). This significant increase became discernible beginning at 8 days after initial exposure. S. exaltata showed slight shoot length reduction at 5.0 μM Pb at 15 days after initial Pb exposure. 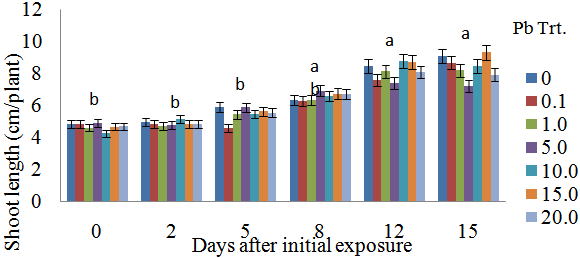 | Figure 4. Shoot lengths of S.exaltata exposed to different Pb concentrations and growth periods. Day means with common letters (on top of bars) do not differ significantly according to LSD test (p < 0.05). Each bar represents the mean + standard error of four replications. |
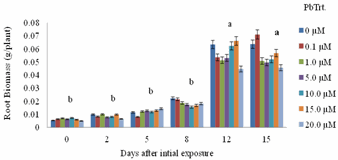 | Figure 5. Root biomass (g/plant) of S. exaltata exposed to different Pb concentrations and growth periods. Day means with common letters (on top of bars) do not differ significantly according to LSD test (p < 0.05). Each bar represents the mean + standard error of four replications. |
Root biomass of S.exaltata increased (p < 0.0004) with exposure to Pb treatments (Figure 5). This increased root biomass became specifically distinguishable at 12 and 15 days post Pb treatment. For each exposure period (e.g., 0, 2, 5, 8 days), there were no significant differences in root biomass regardless of Pb treatments. However, at 12 and 15 days after initial Pb exposure, root biomass of S. exaltata was drastically reduced especially at 20 μM Pb compared to the untreated (0 μM Pb) plants.Similar to root biomass, root lengths of S. exaltata progressively increased with longer exposure periods (Figures 6 and 7). Marked increases in root lengths occurred at 8 days after initial Pb exposure. Generally, for each exposure period, root lengths of Pb-treated S.exaltata were significantly shorter than the untreated plants. However, there were no differences in root lengths regardless of Pb treatments at 15 days post exposure.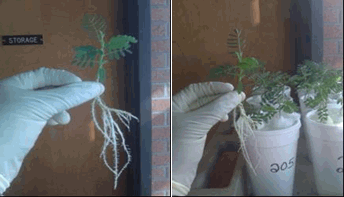 | Figure 6. Comparative root lengths of control (left) and 20 μM treated (right) S. exaltata at 15 days post Pb treatment. |
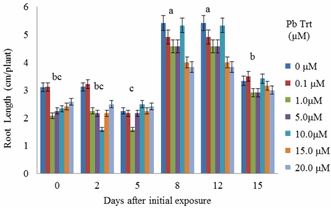 | Figure 7. Root lengths (cm/plant) of S.exaltata exposed to different Pb concentrations and growth periods. Day means with common letters (on top of bars) do not differ significantly according to LSD test (p < 0.05). Each bar represents the mean + standard error of four replications. |
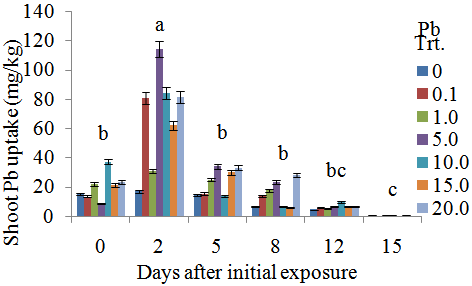 | Figure 8. Shoot Pb uptake of S. exaltata exposed to different Pb treatments and growth periods. Day means with common letters (on top of bars) do not differ significantly according to LSD test (p < 0.05). Each bar represents the mean + standard error of four replications. |
For each Pb exposure period (e.g., 2, 5, 8 days), shoot lead uptake tended to increase as the concentration of Pb treatment was increased (Figure 8). Maximal shoot Pb uptake was exhibited by plants treated with 5 μM Pb on day 2. There were no differences in shoot Pb uptake at 12 days after initial exposure regardless of Pb treatments. Also, there were no appreciable shoot Pb detected at 15 days after initial Pb exposure, indicating minimal or non-existent Pb translocation from the roots to shoots. Marked increases in root Pb uptake (Figure 9) were exhibited by plants exposed to the two highest Pb treatments (e.g., 15 and 20 μM Pb).Generally, root Pb uptake of each exposure period increased with increasing concentrations of Pb treatment (Figure 9). 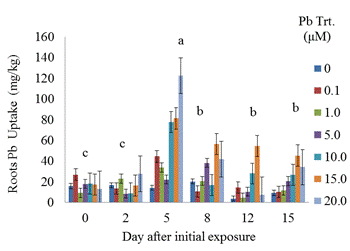 | Figure 9. Root lead uptake of S. exaltata exposed to different Pb treatments and growth periods . Day means with common letters (on top of bars) do not differ significantly according to LSD test (p < 0.05). Each bar represents the mean + standard error of four replications. |
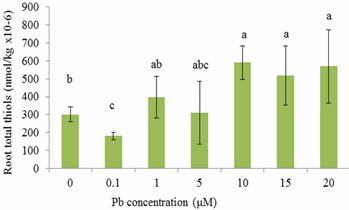 | Figure 10. Total acid-soluble thiols of S.exalatata roots exposed to various Pb treatments at 15 days after initial exposure. Treatment means with common letters are not significantly different according to LSD test (p < 0.05). |
The highest concentrations of total acid-soluble thiols in S. exaltata roots exposed to various Pb treatments were observed on day 10 (Figure 10). Untreated roots had 0.000301nmol/kg of total acid soluble thiols compared to 0.000517 nmol/kg synthesized in roots exposed to 10 μM Pb.There were no significant differences in total acid-soluble thiols synthesized in shoots of S. exaltata that were exposed to different Pb treatments for 15 days (Figure 11). The highest concentration was in plants exposed to 5 μM Pb, wherein they synthesized 0.000344 nmol/kg of total acid-soluble thiols.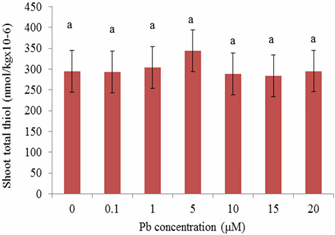 | Figure 11. Total acid-soluble thiols of S. exaltata shoots exposed to various Pb treatments at 15 days after initial exposure. Treatment means with common letters are not significantly different according to LSD test (p < 0.05). |
5. Conclusions
This study demonstrated a controlled laboratory experiment using Sesbania exaltata for accumulating lead. This experiment was performed in the absence of sedimentary material (hydroponic conditions) to evaluate Pb availability more effectively. Results showed that Sesbania exaltata has the potential to be a suitable phytoextraction species. Plants that survived were tolerant to lead except those exposed to 15 μM Pb which exhibited slight leaf abscission and browning of the root system. The greatest metal uptake was observed in the roots. Minimal amounts of lead were translocated to the above-ground plant parts. We can conclude that lead has a low solubility, and metabolic pathways were hampered. Although the plants exhibited some abscission, they displayed tolerance to lead contamination. Among the common pollutants, Cd and Pb activate phytochelatin synthesis as indirectly indicated through the accumulation of acid - soluble thiols. However, binding capacities vary with each metal and lead does not bind as well as Cd. The phytochelatin synthesis was triggered at 10 μM Pb (roots) and 5 μM Pb (shoots) after 15 days of metal exposure. One of the key aspects is that in the laboratory scale, plants are grown in hydroponic controlled environments and are usually exposed only to specific metal treatments.Although the results are encouraging, solution culture is vastly dissimilar from that of soil. In soil, many metals are secured in insoluble forms, and are less accessible. The next step in our search for Pb accumulating plants is to evaluate a wider array of potential hyperaccumulating plants under hydroponic conditions and soil medium. Selected recommendations for future research may include the aid of a chelating agent within a hydroponic solution. Another recommendation would be to test different species of plants simultaneously. While observing the biomass of the plant, a subsequent alternative would be to develop cultivars of plants via biotechnology. This technology could offer a wealth of clones for biochemical, molecular, and physiological assessments of phytochelatin synthesis. There is also a need to examine metal-mobilizing substances called phytosiderophores, which enhance metal uptake.
ACKNOWLEDGEMENTS
This publication was made possible by support provided by the U.S. National Aeronautics and Space Administration (NASA) through The University of Mississippi (UM Subcontract No. 11-08-010 to Jackson State University; M.Begonia, P. I.) under the terms of Agreement No. NNG05GJ72H. The opinions expressed herein are those of the authors and do not necessarily reflect the views of NASA or The University of Mississippi. We acknowledge the partial financial support of the Department of Biology, CSET, JSU.
References
[1] | Leblebici, Z. 2010. Growth and lead accumulation capacity of Lemna minor and Spirodela polyrhiza (Lemnae): Interactions with nutrient enrichment. Water Soil Pollut. 214: 175-184. doi: 10.1007/s11270-010-0413-.1 |
[2] | Sharma, P., and Dubey, R. S. 2005. Lead toxicity in plants. Brazilian J. Plant Physiol. 17(1):35-52 doi:10.1590/S1677-04202005000100004. |
[3] | Lone, M. I. 2008. Phytoremediation of heavy metal polluted soils and water: progresses and perspectives J. of Zhejiang Univ. Sci. (9)3:210-220. Retrieved March 27, 2011, from http://www.zju.edu.cn/jzus/article.php?doi=10.1631/jzus.B0710633. |
[4] | Melegy, A. 2010. Adsorption of lead (II) and zinc (II) from aqueous solution by bituminous coal. Geotech. Geol. Eng., 28(4), 549-558. doi: DOI 10.1007/s10706-010-9309-5 |
[5] | Peralta-Videa, J. R., Lopez, M. L., Narayan, M., Saupe, G., and Gardea-Torresdey, J. 2009. The biochemistry of environmental heavy metal uptake by plants: Implications for the food chain. The Int. J. Biochem. & Cell Biol. 41(9): 1665-1677. doi: 10.1016/j.biocel.2009.03.005. |
[6] | Butcher, D. 2009. Phytoremediation of lead in soil: recent applications and future prospects. Appl. Spectroscopy Rev., 44(2): 123-139. doi: 10.1080/05704920802352580 |
[7] | ATSDR .2007. CERCLA Priority list of hazardous substances. ATSDR Home. Retrieved March 22, 2011, from http://www.atsdr.cdc.gov/cercla/07list.html. |
[8] | Gallardo-Williams, M., Whalen, V., Benson, R., and Martin, D. 2002. Accumulation and retention of lead by cattail (Typha domingensis), hydrilla (Hydrilla verticillata), and duckweed (Lemna obscura). J. Environ. Sci. Health, Part A 37(8): 1399-1408. doi: 10.1081/ESE-120013265 |
[9] | Chaney, R. L., and Malik, M. 1997. Phytoremediation of soil metals. Curr. Opinions Biotechnol. 8: 279-284 |
[10] | Raskin, I., Smith, R. D., and Salt, D. E. 1997. Phytoremediation of metals: Using plants to remove pollutants from the environment. Curr. Opinion Biotechnol. 8: 221-226. |
[11] | Chaney, R. L., Angle, J. S., Broadhurst, C. L., Peters, C. A., Tappero, R. V., and Sparks, D. L. 2007. Improved understanding of hyperaccumulation yields commercial phytoextraction and phytomining technologies. J. Environ. Quality 36(5): 1429-1443. doi: 10.2134/jeq2006.0514. |
[12] | Keltjens, W. G., and Van Beusichem, M. L. 1998. Phytochelatins as biomarkers for heavy metal toxicity in maize: Single metal effects of copper and cadmium. J. Plant Nutr. 21(4): 635-648. doi: 10.1080/01904169809365431 |
[13] | Ellman, G. 1959. Tissue sulfhydryl groups. Arch. Biochem. Biophys. 82(1):70-77. doi: 10.1016/0003-9861(59)90090-6. |
[14] | Anderson, M.E. 1985. Tissue glutathione. Pp. 317-323. In: R. Greenwald (ed.) CRC Handbook of Methods for Oxygen Radical Research. CRC Press, Boca Raton, Florida. |
[15] | U. S. Environmental Protection Agency. 1990. Test methods for evaluating solid wastes. EPA SW-846, EPA, Washington D. C. |