Carissa Mason, Rachel Hjerpe, Emily Noonan, Philip L. Leopold, Antonio Valdevit
Stevens Institute of Technology, Department Biomedical Engineering, Chemistry and, Biological Sciences, Hoboken, USA
Correspondence to: Antonio Valdevit, Stevens Institute of Technology, Department Biomedical Engineering, Chemistry and, Biological Sciences, Hoboken, USA.
Email: |  |
Copyright © 2016 Scientific & Academic Publishing. All Rights Reserved.
This work is licensed under the Creative Commons Attribution International License (CC BY).
http://creativecommons.org/licenses/by/4.0/

Abstract
A combination of mechanical and chemical signals govern tissue responses. Examination of the individual cellular response to the mechanical and the chemical stimuli is essential to understanding these tissue responses. The objective of this study was to develop and optimize a device enabling examination of cellular response under ligand-free compressive loading. This procedure will elicit cellular responses to mechanical loading without confounding biochemical signals imparted by tethering proteins. Particular loading parameters such as amplitude, duration, duty cycle, and frequency require investigation as they likely play a role in mechanotransduction control. We achieved cell nucleus loading through control of paramagnetic microspheres using magnetic fields generated by a hollow solenoid encircling a microscope objective. We placed paramagnetic microspheres upon A549 lung carcinoma epithelial cells in a coverslip dish with a current applied to a solenoid located below the dish. The magnetic field drew the paramagnetic microspheres towards the cells, resulting in nucleus deformation. We captured and analyzed images for morphological changes of the cell nuclei before and during the loading cycle. The results of this study represent the proof of concept for a ligand-free single-cell mechanical loading device for application of compressive loads using paramagnetic microspheres. Applications of this technology include studies involving effects of compressive loading on cell growth as well as investigations into the efficacy of therapeutic drugs when presented to diseased cells.
Keywords:
Ligand-free, Compression, Magnetic microspheres
Cite this paper: Carissa Mason, Rachel Hjerpe, Emily Noonan, Philip L. Leopold, Antonio Valdevit, A Device for Ligand-Free Single Cell Loading Using Magnetic Fields and Paramagnetic Microspheres, American Journal of Biomedical Engineering, Vol. 6 No. 6, 2016, pp. 159-165. doi: 10.5923/j.ajbe.20160606.01.
1. Introduction
According to Wolff’s Law, under compressive loading, bone cells will remodel in response to mechanical stimulation. However, the local environment experienced by bone cells is unknown. Applications of physical forces induce expression of genes, proliferation and, play a fundamental role in regulation of structure, and function within tissues [1]. No reports of studies with respect to cellular response characteristics under ligand-free loading are in the literature. Reported studies using paramagnetic microspheres to apply mechanical forces to osteoblastic integrin are available. However, there is a paucity of work where cellular structures receive direct application of load. Furthermore, the literature is devoid of studies involving mechanical loading of the cells without the use of binding ligand intermediaries.To date, research associated with cellular mechanics and the corresponding mechanotransduction predominately involves the use of ligands and integrins. Several studies utilizing magnetic microspheres to impart loading stimulation to analyze cell response have employed magnetic microspheres attached to integrin receptors [2]. Cells respond to these ligands through the initiation of biochemical signaling pathways, activating enzymes and altering the electrodynamics of the cell membrane. The resulting signals observed may be a sequence containing specific mechanical indicators [3]. Many ion channels expressed in bone cells such as L-type and T-type calcium channels, and potassium channels, are sensitive to these mechanical cues involving ligands. Mechanical loading employing ligands in-vitro increases bone cell proliferation and matrix production. It has also lead to increased bone formation in-vivo [4]. Magnetic microspheres can apply two types of forces to cellular networks: magnetic drag to apply translational or shear forces, and magnetic torque to apply rotational moments. However, both methods require attachment of magnetic microspheres via membrane ligand-receptor interactions, resulting in a signal response that is a combination of the receptor-specific signals and the resulting mechanical stimulation [5].The solenoid configuration in conjunction with the microscope permits visualization of ligand free loading through use of paramagnetic microspheres. This initial study represents the first report of compressive loading imparted upon cells without the use of binding proteins. We hypothesized that a significant mechanical response to compressive loading is achievable through ligand-free induced loading by paramagnetic microspheres in magnetic fields.
2. Materials and Methods
To induce a magnetic field in the hollow solenoid a programmable DC power supply (1785B, BK Precision, Yorba Linda, CA) provides pre-selected current profiles. The solenoid is self-contained and designed to circumvent a microscope objective. This feature permits the ability to observe and capture images of the resulting cellular morphological changes due to compression from magnetic microspheres directly upon cellular surfaces without the use of ligand binding proteins.
2.1. Device Design and Modification
In order to increase magnetic field strength, a solenoid core with flanges on the top and bottom permits layering of coils. The solenoid possessed 240 turns in six layers of 40 turns each. The solenoid design is slightly larger than the diameter (41.3 mm) of the microscope objective, allowing for better heat dissipation during operation in contrast to the previous design. Removing solenoid heating was crucial so as not to influence morphological changes due to thermal effects. Figure 1 illustrates the solenoid design.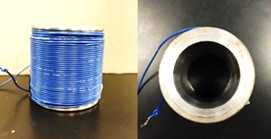 | Figure 1. Side and top view of solenoid design |
2.2. Optimization of Function Generator
To ensure the effective movement of the magnetic microspheres, different magnetic microsphere suspensions in deionized (DI) water were prepared. We performed an experimental procedure to maximize microsphere movement visibility and determine optimized concentration of magnetic microsphere suspension for the most rapid solution clearance. At a 5mm distance above the solenoid, we placed a glass pipette containing 1ml of magnetic microsphere suspension. A permanent magnet located above the pipette. The permanent magnet is required as the combination of microsphere agglutination, adhesion to the cell surface and, the presence of the earth’s magnetic field would maintain the magnetic microspheres in contact with the cell surface resulting in continuous compression. As well, presence of these permanent magnets serves to retard the approaching velocity of the magnetic microspheres (due to the solenoid) as they contact the cells. Furthermore, the permanent magnets draw the magnetic microspheres back into suspension for unloading of the cells. This is essential during the rest component of the duty cycle and unloading portions of dynamic loading regimens. The programmable DC power supply applied a solenoid current between 0-5A at 0-15V. Figure 2 illustrates the experimental configuration for determination of magnetic microsphere suspension movement.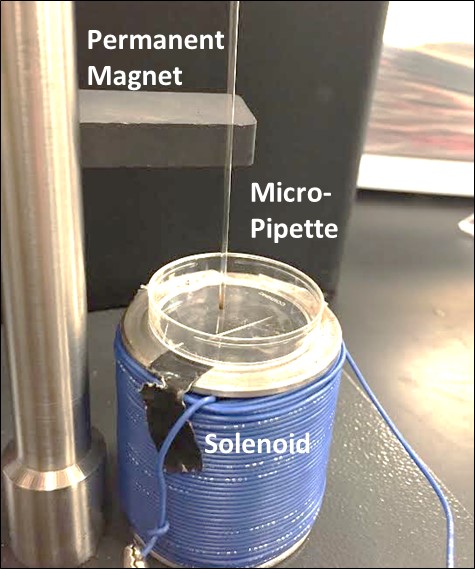 | Figure 2. We placed a petri dish above the solenoid. Permanent magnets above the dish prevented the microspheres from affixing to the bottom of the dish due to microsphere agglutination, surface adhesion, and the earth’s magnetic field |
We examined suspensions with magnetic microsphere to DI water ratios of 1:2, 1:4, 1:8, 1:10 1:16, and 1:20 using 1μm diameter paramagnetic microspheres (Spherotech, Inc., Lake Forest, IL). Additionally, we performed experiments with varying numbers of permanent magnets secured to the top of the pipette with the purpose of retarding the microsphere velocity upon cellular contact.Solenoid operation consisted of a 25% duty cycle with 10 seconds of power and 30 seconds of cooling during application of 10V and 1.85A. Such a configuration was sufficient to keep the solenoid from overheating. Under these experimental conditions, we recorded the time required for clearance of the various magnetic microsphere concentrations within the glass pipette containing 1ml of magnetic microsphere suspension.
2.3. Cell Culture
For proof of concept testing, we grew A549 lung epithelial carcinoma cells (ATCC, Manassas, VA) in culture flasks in F12K (10% FBS Atlanta Biologicals, Flowery Branch, GA, 1% PBS, Gibco, Life Technologies, 1% Fungizone, Gibco, Life Technologies) cell culture medium at 1:10 concentration. We performed sub-culturing of cells every four days and exchanged media every two days. Prior to splitting, we warmed the culture medium to 37°C and subsequently split the cells by removing media and washing with 8mL of PBS. We then added 2ml of 0.05% trypsin to the culture flask and incubated for 5min. To halt the action of trypsin, we added 8ml of warm culture media to the flask following the incubation period. To 9m of fresh media, we added 1ml of cells from the flask.For microscope imaging, we transferred cells to 37mm diameter coverslip-bottomed dishes. We stained the cells by adding 10μl Hoechst 33324 stain to the media followed by 30min of setting time at which point we removed all media of the coverslip dish except for the center well. Prior to introduction with the cells, the magnetic microspheres were washed in OptiMEM culture medium (1X reduced serum medium, Gibco, Life Technologies) in order to remove sodium azide, which was present in the original microsphere suspension. We added 0.2ml of the original microsphere solution to a centrifuge tube with culture media added for a total volume of 1.5ml and centrifuged at 6000 RPM for 3min. We removed the supernatant and replaced the volume with OptiMEM medium with centrifugation repeated three times. We vibrated the tube to distribute the microspheres throughout the solution prior to extracting 50μl of microsphere solution for placement atop the cells in the coverslip dish.
2.4. Compressive Loading and Imaging
We performed compression experiments to analyze movement of nuclei within the cells due to applied loads from the paramagnetic microspheres. We placed permanent magnets superior to the cells on top surface of the coverslip dish to re-draw the paramagnetic microspheres to the top of the culture medium. This effectively removes compressive loading due to the microsphere contact with the cells while the solenoid is off. We placed the solenoid around the 40X ELWD (Extra Long Working Distance) objective of a Nikon Eclipse TE2000-U microscope. We isolated locations within the coverslip dish with high volumes of microspheres and examined cells for areas where microspheres were located directly on the surface of the cells. We identified these locations as focal points for investigation when we were able to observe interior structures. We imaged the paramagnetic microspheres, cells, and cell nuclei before and after loading using a Nikon Intensilight C-HGFI camera. Using NIS Elements Advanced Research Microscope Imaging Software, we viewed and overlaid the images. Fluorescence images of cell nuclei before (red and green) and during (blue and green) loading were overlaid and subsequently analyzed for changes in nuclear morphology. We selected 10 locations for analysis within each dish. Figure 3 depicts the schematic and experimental set-up.We captured cell nuclei images before and during each cycle of loading with images of the unloaded nuclei recaptured following the termination of power to the solenoid. We then resumed compressive loading after 30s for a second time with the imaging process repeated. Generation of images overlays included all images: unloaded versus first loading cycle, first loading cycle versus unloading, and the second unloaded cycle versus the second application of loading. More specifically, fluorescence images of nuclei before and after loading or before and after the absence of loading were loaded into green and red channels, respectively. Areas of the image that were unchanged before and after loading appeared yellow while areas of the image that changed due to loading appear green if present only before loading and red if present only after loading. To create composite images of nuclei and beads, nuclear images were loaded into red and green channels while brightfield images of beads were loaded into the blue channel. In some cases, a single nuclear image was loaded into the green channel and was summed with the brightfield bead image to create an image with cyan nuclei overlaid with the blue brightfield image of beads. An alternative means of demonstrating the change in nuclear morphology before and after loading was to use the “ratio imaging” function in the Nikon Imaging System software to compare two monochrome images yielding a tricolor map of image differences. In this case, pixel intensity instead of simply pixel position revealed the shift in nuclear position under loading.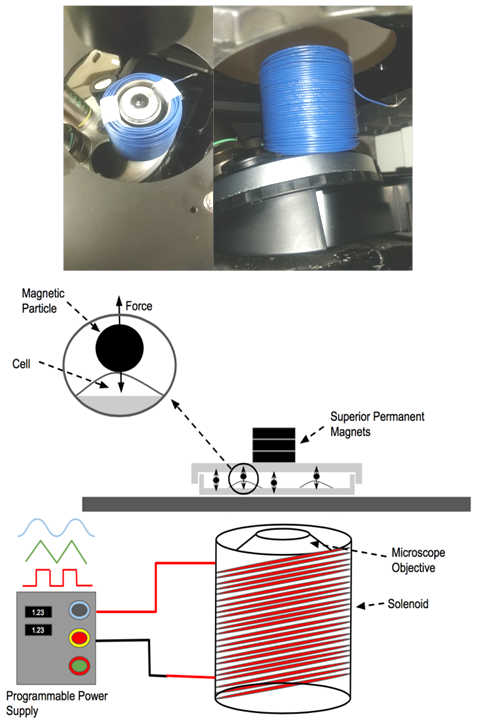 | Figure 3. Depiction of the schematic and experimental set-up for application of mechanical loading to cells using paramagnetic microspheres. The magnetic field generated by a solenoid encircling an optical microscope objective |
The Major and Minor axes of the cell nuclei are computed by measuring the number of pixels across the nucleus. We selected the initial and final points for measurement as the location where pixels displayed a change from bright blue on the edge of the cell to darker blue around the cell. We apply this process to the pixel length determination for both the Major and Minor cell nucleus axis. Figure 4 illustrates the major and minor axes computed (left) as well as the change in blue hue used as the edge determination for the respective axes (right). The margin of error for these calculated measurements are within four pixels or ±2.67μm.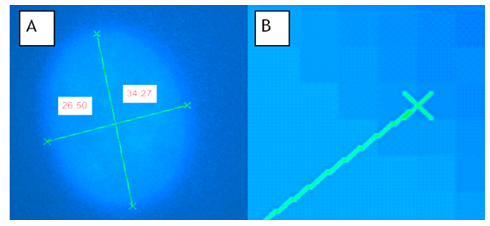 | Figure 4. [A] Measurement of axes. [B] The criteria for the start and end of the respective axis was taken as the points at which pixels began fading from the bright blue on the edge of the cell to the darker blue around the cell |
3. Results
3.1. Function Generator Optimization
We selected a 1:20 ratio of magnetic microspheres to DI water as the optimal solution for both microsphere movement visibility and microsphere movement time. Ratios with increased magnetic microspheres relative to the amount of water required larger time intervals to move all of the microspheres through the solution. Table 1 displays the microsphere migration times for various microsphere-DI water solutions.Table 1. Time (in seconds) for microspheres to migrate 1mm in the presence of one, two, and three superiorly placed permanent magnets 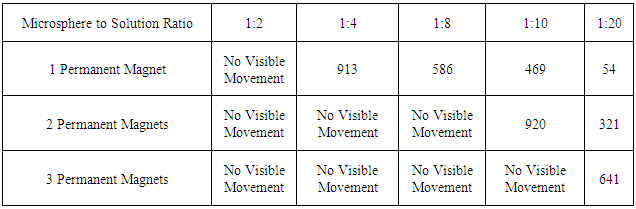 |
| |
|
We also found that the increased time intervals with the solenoid in operation significantly influenced the heating within the solenoid coils. We selected a microsphere to water ratio of 1:20 was selected as it was experimentally determined to be the greatest concentration of magnetic microspheres that could be employed without resulting in overheating the solenoid due to prolonged operation time. In the loading experiments, use of the 1:20 microsphere to water ratio and the 10s on 30s off duty cycle condition minimized heating of the solenoid coils.
3.2. Cellular Response to Loading
We applied 5 cycles of loading to analyze physical changes within the A549 lung epithelial cells with the last two cycles used for data acquisition. During loading regimens, we captured fluorescent images of cell nuclei before and during loading. Figure 5 (A, B) illustrates observed differences in microsphere locations between images.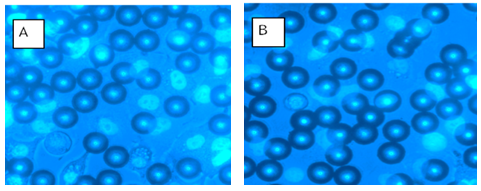 | Figure 5. Representative examples of high-density microsphere and nuclei locations chosen for examination. [A] Prior to loading (solenoid off) and [B] Active loading (solenoid on). Microspheres are darker in B as they are closer to the microscope objective due to the magnetic field generated by the solenoid |
For comparison, we overlaid the images in blue and green, respectively. Areas of blue and green along the edges of cell nuclei were indicative of nuclear movement due to mechanical loading (Figure 6).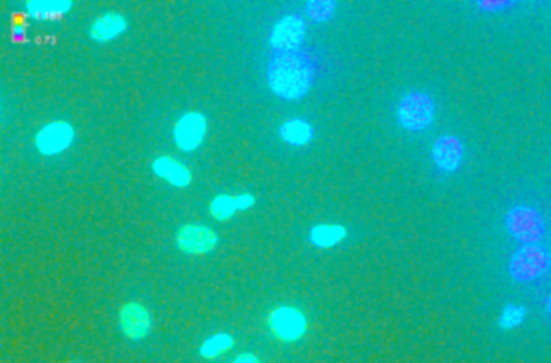 | Figure 6. The merged image between two images: solenoid off and solenoid on without displaying of the magnetic microspheres. The blue edges show a morphology shift due to compressive microsphere loading due to the generated magnetic fields |
We overlaid images of the nuclei captured before and during loading to reveal movement as depicted in images C and E of Figure 7. It is important to note that the presence of the function generator and magnetic fields created by the solenoid did not interfere with function of the microscope or image acquisition.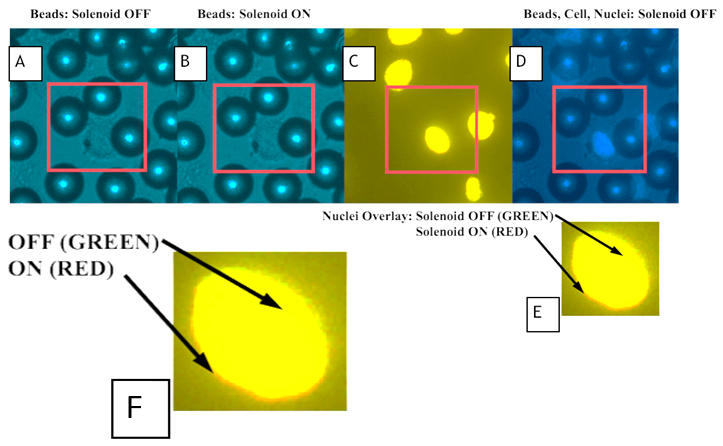 | Figure 7. Single cell loading using paramagnetic microspheres. [A] Microspheres and cells with solenoid off, [B] Microspheres and cells with solenoid on, [C] Overlay of cell nuclei for solenoid on vs solenoid off, [D] Microspheres and cell nuclei for solenoid off, and [E] Magnified image of overlaid cell nuclei for solenoid off (green) vs. solenoid on (red), [F] Magnified view of [E] illustrating “green” and “red” overlay indicative of nuclear dimensional changes |
Student’s paired t-tests were performed to compare the respective Major and Minor axis distances of cell nuclei prior to and under compressive magnetic loading with P<0.05 for statistical significance. We repeated the imaging and axis computation regimen under conditions where paramagnetic microspheres were not located near cells. We performed this process to ascertain if cellular morphology changes could be due to thermal effects from the heating of the solenoid coils. The same statistical analysis was conducted at six cell locations where microspheres where not located upon or near a cell. We obtained images at these locations with and without power to the solenoid and computed the Major and Minor axis distances for each image as previously described.In the case of magnetic microsphere compression, no statistically significant differences (P>0.6) in the nucleus Minor axis dimension was detected when cells were subjected to loading. However, in the measurement of the Major axis dimension of the nucleus, a statistically significant difference was seen when cells were subjected to microsphere compression (P<0.01) (Figure 8).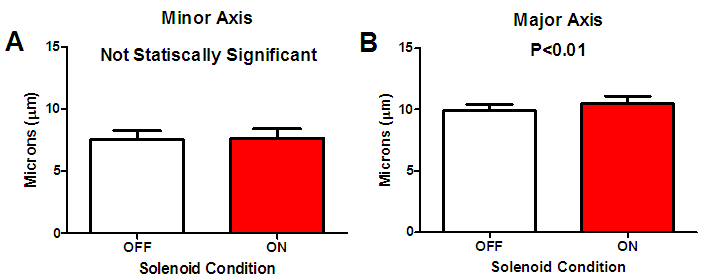 | Figure 8. Changes in cell nucleus dimensions due to magnetic microsphere loading [A] Minor axis (P>0.7) and [B] Major axis (P<0.01) |
Direct conversion to strain using the pixel to micron scaling resulted in an applied loading strain in the Minor axis of 1.3% while the Major axis displayed a strain of 5.6%. We also computed cell nucleus dimensional changes in cases where magnetic microspheres are absent (Figure 9, A, B). A non-significant P value (P>0.08) revealed that the thermal effects of the solenoid coils did not influence the morphological response observed. Thus, magnetic fields generated when the solenoid is in operation is the source of morphological changes only when magnetic microspheres are imparted upon the cellular surface leading to compressive forces being generated.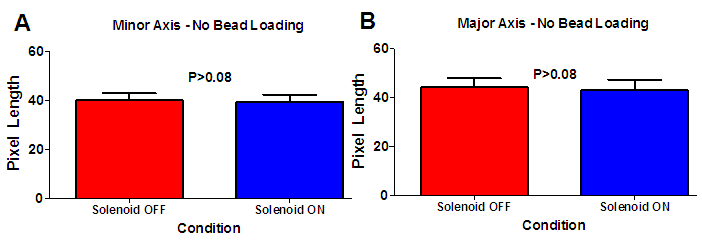 | Figure 9. Changes in cell nucleus distance due to the presence of the solenoid without the presence of magnetic microspheres. [A] Minor axis [B] Major axis changes due to solenoid operation were not statistically different (P>0.08 for both) |
4. Discussion
In this study, we have demonstrated the successful use of varying magnetic fields to induce compressive forces via paramagnetic microspheres. Observation of a statistically significant increase in the Major axis length of the loaded cell nuclei as compared to the unloaded condition is noted. In addition, there are no statistically significant results in morphology changes of the cell nucleus due to the solenoid operation in cases where magnetic microspheres were not present upon the cellular surface. Thus, the changes observed were the result of direct compressive loading induced by the paramagnetic microspheres and not the result of thermal effects due to solenoid operation. This work has established the framework for a portable and economical device for application of loading conditions in biological environments to study cellular response under ligand-free conditions.The device developed is applicable for controlled single-cell loading to isolate the biological response of cells to mechanical activation. Since the microspheres are not bound to the cell via ligands, use of superiorly positioned permanent magnets permits the possibility of unloaded conditions. Continuous periodic, ligand-free loading represents a physiological loading regimen as compared to static, mechanical, ligand-selective stimulation. Biological evaluations of different cells to applied loading are possible with the magnetic device and experimental procedures developed. The microspheres utilized in this study are sufficiently large to prevent internalization by the cells subjected to loading thereby permitting performance of long-term stimulation experiments.In this experiment cells experienced mechanical compression due to the attraction of the paramagnetic microsphere (residing upon the cellular surface) by the magnetic field generated by the solenoid. We recorded strain values of 1.3% for the Minor axis of the cell nucleus while we found a strain level of 5.6% in the direction of the Major axis. Sen et.al., applied daily regimens of mechanical strain to mesenchymal stem cells (MSCs) and recorded an inhibited expression of peroxisome proliferator-activated receptor γ and adiponectin mRNA suggesting that mechanical strain alone can affect cell differentiation. Regimens consisted of daily applications of 2% strain [6]. The operation of this device is certainly capable of applying such levels of mechanical stimulation as we can adjust strain levels through variations in solenoid current and number of solenoid wire turns.Paramagnetic microspheres are effective for the delivery of highly localized mechano-inductive forces directly to cells [7]. The advantage of using paramagnetic microspheres in such an approach has been the ability to apply force directly to cells rather than transmitting forces through a scaffold or other intermediary mechanism [8, 9]. Forces applied directly to the cell rather than the scaffold eliminates mechanical restrictions that are commonly present. The non-invasive nature of such loading also eliminates the need for complex machinery to apply forces to cells, hence, improving the sterility of such studies [10]. Upcoming studies will focus on the response of osteoblasts to single cell ligand-free compressive loading, in contrast to studies where integrin attachment to magnetic microspheres a lead to changes in intracellular calcium signaling within osteoblasts [11]. Despite the wide acceptance of the sensitivity of bone formation and resorption sensitivity to mechanical demands, there remains uncertainty regarding the chemical messengers produced in response to mechanical loading [12]. The device created in this study could be beneficial in pursuing further research on this topic. Through application of highly localized, ligand-free loading, studies of changes in several different cell signaling molecules can be performed to better understand the biological changes within the cell along with the observed physical changes.
5. Conclusions
We have demonstrated a proof of concept for a single cell compressive loading device using paramagnetic microspheres. Forces applied using paramagnetic microspheres resulted in physical morphological changes within the cell nuclei. The device created will better enable research into the biological responses of cells to mechanical loading. In the future, the use of this device to permit localized delivery of mechanical loading or experimental drug delivery without confounding ligand binding could be very beneficial in the orthopedics and pharmaceutical industries. Cellular morphological changes due to magnetic loading conditions resulted in a statistically increased Major axis dimension of the cell nucleus. Combined with a statistically equivalent morphological result due to magnetic field application without the use of magnetic microspheres, one can conclude that the geometrical changes observed are entirely due to the magnetic microsphere loading alone. This study presents a ligand free single cell-loading device employing paramagnetic microspheres.
References
[1] | Yuge L, Okubo A, Miyashita T, Kumagai T, Nikawa T, Takeda S, Kanno M, Urabe Y, Sugiyama M, and Kataoka K. Physical stress by magnetic force accelerates differentiation of human osteoblasts. Biochemical and Biophysical Research Communications. 2003; 311: 32–38. |
[2] | Davidson R, Tatakis D, and Auerbach A. Multiple forms of mechanosensitive ion channels in osteoblast-like cells. European Journal of Physiology. 1990; 416(6): 646-651. |
[3] | Hughes S, El Haj AJ, Dobson J. Magnetic micro- and nanomicrosphere mediated activation of mechanosensitive ion channels. Medical Engineering & Physics. 2005; 27: 754–762. |
[4] | Lessey EC, Guilluy C, Burridge K. From mechanical forces to RhoA activation. Biochemistry. 2012; 51 (38): 7420-7432. |
[5] | Vance J, Galley S, Liu D, and Donahue S. Mechanical stimulation of MC3T3 osteoblastic cells in a bone tissue-engineering bioreactor enhances prostaglandin E2 release. Tissue Engineering. 2006; 11(11): 1832-1839. |
[6] | Sen B, Xie Z, Case N, Ma M, Rubin C, Rubin J. Mechanical Strain Inhibits Adipogenesis in Mesenchymal Stem Cells by Stimulating a Durable β-Catenin Signal. Endocrinology. 2008; 149:12; 6065–6075. |
[7] | Riggio C, Calatayud MP, Hoskins C, Pinkernelle J, Sanz B, Torres TE, Ibarra MR, Wang L, Keilhoff G, Goya GF, Raffa V, Cuschieri A. Poly-l-lysine-coated magnetic nanomicrospheres as intracellular actuators for neural guidance. 2012; Int J Nanomedicine. 7: 3155–3166. |
[8] | Berry C Catherine, Curtis Adam. Functionalism of magnetic nanomicrospheres for applications in biomedicine. IOP Science. 2003; 36: 13; 202. |
[9] | Bock N, Riminucci A, Dionigi C, Russo A, Tampieri A, Landi E, Goranov VA, Marcacci M, Dediu V. A novel route in bone tissue engineering: magnetic biomimetic scaffolds. Acta Biomater. 2010; 6(3): 786-96. |
[10] | Yuge L, Okubo A, Miyashita T, Kumagai T, Nikawa T, Takeda S, Kanno M, Urabe Y, Sugiyama M, and Kataoka K. Physical stress by magnetic force accelerates differentiation of human osteoblasts. Biochemical and Biophysical Research Communications. 2003; 311:32–38. |
[11] | Hughes S, Dobson J, El Haj AJ. Magnetic targeting of mechanosensors in bone cells for tissue engineering applications. Journal of Biomechanics. 2007:40; S96–S104. |
[12] | Glogauer M, Ferrier J. A new method for application of force to cells via ferric oxide microspheres. Pflugers Arch. 1998; 435(2): 320-7. |