Carla Cunha1, 2, Silvia Panseri1, 2, Maurilio Marcacci1, Anna Tampieri2
1Laboratory of Biomechanics and Technology Innovation, Rizzoli Orthopaedic Institute, Bologna, 40136, Italy
2Laboratory of Bioceramics and Bio-hybrid Composites, Institute of Science and Technology for Ceramics, National Research Council, Faenza, 48018, Italy
Correspondence to: Silvia Panseri, Laboratory of Biomechanics and Technology Innovation, Rizzoli Orthopaedic Institute, Bologna, 40136, Italy.
Email: |  |
Copyright © 2012 Scientific & Academic Publishing. All Rights Reserved.
Abstract
There is a general interest in the effects of magnetic fields on human tissue, for biomedical imaging, cancer therapy or tissue engineering applications. In particular the orthopaedic field may greatly benefit from magnetic scaffolds, magnetic fixation and magnetic delivery techniques. In this study, MG-63 osteoblast-like cells were analysed in vitro after exposure to a 320mT static magnetic field (SMF), either continuously or applied for 1h at any 24-hour interval. Results demonstrate that SMF causes a reduction in cell number after 7 days of exposure, as demonstrated by the MTT assay. This reduction in proliferation is not associated to an increase in Lactate Dehydrogenase production, a marker of cellular stress and/or disruption of membrane integrity. Osteocalcin secretion increased at day 3 for the condition 1h/day exposure and this effect was reversed after 7 days. Instead, the continuous application of a SMF resulted in a significantly decreased osteocalcin release at day 7. Cell distribution, morphology and cytoskeleton organization were unaltered, with the typical osteoblastic morphology maintained and normal distribution of cytoplasmic actin fibrils, as demonstrated by phalloidin staining. Gene expression analysis of COL1A1, ALPL and RUNX2 show no alterations respect to control. These results suggest that such a moderate intensity static magnetic field has a detrimental effect on cell proliferation and osteocalcin secretion, while maintaining morphological features and gene expression unaltered. The in vitro effects of magnetic fields depend on cell type, magnetic field intensity and modality of application. This study gives a contribution to understand moderate strength static magnetic field effects on human tissue, with particular importance for the orthopaedic field.
Keywords:
Static Magnetic Field, Osteoblast Behaviour, Gene Expression, Magnetic Scaffolds
Cite this paper:
Carla Cunha, Silvia Panseri, Maurilio Marcacci, Anna Tampieri, "Evaluation of the Effects of a Moderate Intensity Static Magnetic Field Application on Human Osteoblast-Like Cells", American Journal of Biomedical Engineering, Vol. 2 No. 6, 2012, pp. 263-268. doi: 10.5923/j.ajbe.20120206.05.
1. Introduction
There has been an increasing interest in the study of magnetic field employment in the clinical setting, either for magnetic resonance imaging, for magnetically guided delivery of biomolecules for tissue engineering applications or cancer therapy. In particular in the orthopaedic field, the use of static magnetic fields finds a broad range of applications, from the ones mentioned to the possibility of having in situ magnetic fixed stations for prosthesis or scaffold fixation. In fact, our laboratory has recently developed a simulated scaffold fixation technique for osteochondral defect surgery that makes use of magnetic forces attracting the scaffold towards the implanted position1. Also the use of superparamagnetic nanoparticles (MNPs) for biological and medical purposes has been increasing, either as contrast agent for diagnostic MRI imaging, for its hyperthermia effect, for magnetic drug delivery and for tissue engineering applications2-6. We have recently demonstrated that intrinsically magnetic iron-substituted hydroxyapatite nanoparticles significantly increase osteoblast proliferation and activity when exposed to a static magnetic field 7 and the same nanoparticles have been used together with a biodegradable poly(caprolactone) core for the development of a magnetic scaffold for bone tissue engineering 8. Also, we have introduced magnetite into the structure of a macroporous hydroxyapatite ceramic scaffold, which demonstrated in vitro biocompatibility, either in the presence or absence of a magnetic field, and good in vivo osteointegration 9. Moreover, we have designed two hydroxyapatite/collagen (70/30 wt %) magnetic scaffolds, magnetized with two different techniques: direct nucleation of biomimetic phase and MNPs on self-assembling collagen fibers and scaffold impregnation in a ferro-fluid solution 10,11. Recently, we have designed magnetic biohybrid scaffolds made of collagen and intrinsically magnetic iron-substituted hydroxyapatite for bone regeneration 12. The possibility of using superparamagnetic phases mostly relies on the application of an external magnetic field able to activate such materials and/or to drive biomolecule loaded MPN to desired specific sites. The effects of such magnetic fields on the human tissue need to be well investigated in order to understand the possible range of application of such magnetic devices and its implication for the different biomedical applications. Cell culture studies directed at exploring both the biocompatibility of magnetic devices and also the effects of magnetic fields in biological tissues are needed. In this context, this study analysed for the first time the effect of a moderate intensity static magnetic field (320 mT) on MG-63 human osteoblast cell culture, and intends to give a contribute to deepen the knowledge on possible applications of magnetic devices driven by magnetic forces in the clinical setting.
2. Materials and Methods
2.1. In Vitro Cell CultureMG-63 human osteoblast-like cells (Lonza, Italy) were cultured in DMEM/F12 medium (PAA, Austria) containing 10% FBS and Penicillin-Streptomycin (100 U/ml-100 µg/ml) and kept at 37℃ in an atmosphere of 5% CO2. Cells were detached from culture flasks by trypsinization, centrifuged and resuspended. Cell number and viability were assessed with the trypan-blue dye exclusion test. 2.5x104 cells per well were plated into a 24-multiwell and cultured for 1, 3 and 7 days, in the absence (control) or presence of an external magnet, which relied on the application of a commercially available static magnetic field of 320 mT under the multiwell plates (MagnetoFACTOR-24, Chemicell, Germany), which ensures equal application of the magnetic field under each well. A third condition relied on the exposure of cells to the static magnetic field for 1 hour at any 24-hour interval. All cell handling procedures were performed in a sterile laminar flow hood. Cell culture incubation was performed at 37℃ with 5% CO2.
2.2. MTT Assay
The MTT reagent (3-(4,5-dimethylthiazol-2-yl)-2,5- diphenyltetrazolium bromide) (Invitrogen) was prepared at 5 mg/ml in 1x PBS. Cells were incubated with the MTT reagent 1:10 for 2 h at 37℃. Medium was discarded and cells incubated with 1 ml of Dimethyl sulfoxide (Sigma) for 15 min. In this assay, the metabolically active cells react with the tetrazolium salt in the MTT reagent to produce a formazan dye that can be observed at λmax of 570 nm, using a Multiskan FC Microplate Photometer (Thermo Scientific). This absorbance is directly proportional to the number of metabolically active cells. Mean values of absorbance were determined. Three samples were analysed per group per time point.
2.3. Lactate Dehydrogenase Assay
Liqui Lactate Dehydrogenase (LDH) assay (Futura System) was performed according to manufacturer's instructions. Briefly, LDH solution was prepared by mixing 4 parts of Buffer solution to 1 part of Substrate solution. 20 µl of cell medium was added to 1 ml of LDH solution. Absorbance was read at 0, 1, 2 and 3 min at λmax of 340 nm, using a Multiskan FC Microplate Photometer (Thermo Scientific). LDH activity was determined by calculating the ∆OD/min. LDH activity was expressed as U/l. Three samples were analysed per group per time point.
2.4. Human Osteocalcin Elisa
Osteocalcin (OST) secretion was measured by the Human Osteocalcin ELISA kit (Invitrogen), following manufacturer's instructions. Briefly, 25 µl of cell medium was incubated with 100 µl of anti-OST-HRP antibody for 2 hours at room temperature, within the supplied microtiter plate, coated with a second anti-OST antibody. After washing, 100 µl of chromogenic solution was added to the wells and plate incubated at room temperature in the dark. Reaction was stopped 25 min after addition of 100 µl of stop solution. Absorbance was read at λmax of 450 nm, using a Multiskan FC Microplate Photometer (Thermo Scientific). The amount of osteocalcin was determined by interpolation from a standard curve of known concentrations. Osteocalcin concentration was expressed as ng/ml. Three samples were analysed per group per time point.
2.5. Phalloidin Immunofluorescence Staining
Cells were washed with 1x PBS for 5 min, fixed with 4% (w/v) paraformaldehyde for 15 min and washed with 1x PBS for 5 min. Permeabilization was performed with 1x PBS with 0.1% (v/v) Triton X-100 for 5 min. FITC-conjugated Phalloidin antibody (Invitrogen) 1:500 in 1x PBS was added for 20 min at room temperature in the dark. Cells were washed with 1x PBS for 5 min and incubated with 300 nM DAPI (4'-6-Diamidino-2-phenylindole) solution (Invitrogen) in 1x PBS for 5 min. Images were acquired by an Inverted Ti-E fluorescence microscope (Nikon). Two samples per group were analysed.
2.6. Total RNA Extraction and Reverse Transcription
At each time point, cells were collected by trypsinization, centrifuged, resuspended in RNAlater solution and kept at -20℃. Total RNA isolation was performed by use of the Tris reagent, followed by the Purelink RNA Mini kit according to manufacturer's instructions. In particular, we have used a on-column DNAse treatment by use of the Purelink DNAse set. Purified total RNA was eluted with THE RNA storage solution and kept at -80℃ until reverse transcription. RNA integrity was analysed by native agarose gel electrophoresis (1.5% agarose in 1x TBE, with 1 µg/ml of ethidium bromide), run in 1x TBE buffer and quantification performed by the Qubit® 2.0 Fluorometer (Invitrogen), together with the Qubit® RNA BR assay kit, following manufacturer's instructions. cDNA was obtained by using the High capacity cDNA reverse transcription kit, according to manufacturer's instructions. In particular, we have used 1.5 µg of total RNA in a 20 µl final reaction volume for each sample, as follows: 2 µl of 10x RT buffer, 0.8 µl of 25x dNTP mix, 2 µl of 10x RT primers, 1 µl of RNAse inhibitor, 3.2 µl of Nuclease free water plus 10 µl of template. Reaction conditions: 25℃ for 10 min, 37℃ for 120 min, 85℃ for 5 min. cDNA was kept at -20℃ until qPCR. All reagents and instruments were from Life Technologies.
2.7. Quantitative Real-time Polymerase Chain Reaction (qPCR)
Quantification of Collagen, type I, alpha 1 (COL1A1), Alkaline Phosphatase (ALPL), Runt-related transcription factor 2 (RUNX2) and glyceraldehyde 3-phosphate dehydrogenase (GAPDH) gene expression was performed by use of the StepOne™ Real-Time PCR System (Applied Biosystems). We used the Taqman gene expression mastermix and the following Taqman gene expression assays: GAPDH: Hs99999905_m1, COL1A1:Hs00164004_m1, ALPL: Hs01029144_m1 and RUNX2:Hs00231692_m1, all with the Fam™ dye as reporter. 1 µl of cDNA was used in a 10 µl final reaction volume, as follows: 5 µl of 2x mastermix, 0.5 µl of 20x gene expression assay, 3.5 µl of Nuclease free water plus 1 µl of template. qPCR reaction was performed on a 48-well plate adhesive sealed, as follows: 40 cycles at 95℃ for 15 sec, 60℃ for 1 min, after an initial denaturation step of 50℃ for 2 min and 95℃ for 10 min. Data was collected using the OneStep Software (Applied Biosystems) and relative quantification was performed using the comparative threshold (Ct) method (ΔΔCt), where relative gene expression level equals 2−ΔΔCt (Livak and Schmittgen, 2001), as described by the manufacturer. In detail, changes in gene expression level of the target genes COL1A1, ALPL and RUNX2 were calculated by normalization to the reference GAPDH and by normalization to the control condition, used as calibrator, within each sample set. Changes in gene expression were reported as fold changes of target relative to controls and represent averages from triplicate measurements. All reagents and instruments used for gene expression analysis were from Life Technologies.
2.8. Statistical Analysis
For MTT, LDH and Osteocalcin experiments, results were expressed as MEAN±SEM plotted on graph (n=3). Analysis of differences between groups was made by one-way ANOVA for each time point, followed by Dunnett's post-hoc test. For gene expression experiments, results were expressed as RQ with MAX and MIN values plotted on graph (n=3). All statistical analysis made use of the GraphPad Prism software (version 5.0), with statistical significance set at p≤0.05.
3. Results and Discussion
In this study, we analysed in vitro the effect on a MG-63 osteoblast culture of a moderate strength static magnetic field (SMF) of 320 mT, a value that is within the range (1mT to 1T) demonstrated to have particular implications in a number of biological phenomena13,14. We have used two different conditions: continuous exposure and exposure for 1h per day, envisaging a possible clinical setting application, where the patient would be submitted to therapy for a restricted period of time. Experimental time points were for all experiments 1, 3 and 7 days, except for gene expression analysis where only day 7 was considered.
3.1. Application of a Static Magnetic Field Reduces Cell Proliferation
The MTT assay is a reliable method to quantify cell proliferation at each time point. Results demonstrate that when exposed for either 1h/day or continuously to a SMF, cells continue to grow from day 1 to day 7 (Fig. 1A), but presenting a considerably reduced number of cells respect to control, at day 7 (p≤0.01). Still, this reduction in proliferation observed at day 7 is not associated to an increase in cellular apoptosis, stress or disruption of membrane integrity, in fact quantification of the cellular stress marker Lactate Dehydrogenase (LDH), released when cell membrane integrity is disrupted, showed no difference between groups at any of the time points (Fig. 1B). 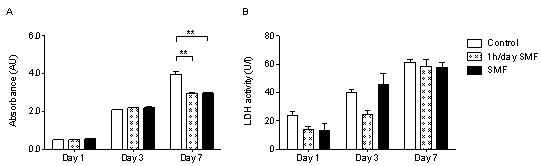 | Figure 1. Analysis of cell proliferation and cellular stress. Quantification of cell proliferation was performed by the MTT assay (A) and quantification of cellular stress was performed by the Lactate Dehydrogenase (LDH) assay (B) at 1, 3 and 7 days for control osteoblasts, osteoblasts exposed for 1h/day to a static magnetic field (SMF) or continuously exposed to a SMF. n=3, ** p≤0.01 |
The observed reduction in proliferation is in contrast to a previous study that used murine MC3T3-E1 osteoblasts and showed that a moderate SMF resulted in enhanced proliferation 13. Still, this was a different cell line and the intensity of the SMF was less than half (150 mT) the one used in this study (320 mT), which could explain different results.Another study instead demonstrated MG-63 osteoblasts increased proliferation when exposed to a 400 mT SMF, yet this study was performed on a poly-L-lactide substrate 15.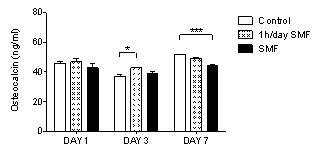 | Figure 2. Analysis of the osteogenic marker osteocalcin. Quantification of released osteocalcin was performed by ELISA at 1, 3 and 7 days for control osteoblasts, osteoblasts exposed for 1h/day to a static magnetic field (SMF) or continuously exposed to a SMF (n=3). * p≤0.05, *** p≤0.001 |
3.2. Osteocalcin is Reduced after 7 Days of Exposure
Osteocalcin is secreted by osteoblasts and is the major non collagenous component of the bone extracellular matrix, being used as a reliable marker for bone formation. The quantification of the osteocalcin released by MG-63 osteoblasts to the cell culture was performed by the Human Osteocalcin Elisa Kit. Results demonstrate that application of a SMF for 1h/day increases osteocalcin release at day 3, respect to control (p≤0.05), while this effect is reversed after 7 days (Fig. 2). Instead, the continuous application of a SMF resulted in decreased osteocalcin release, respect to control (p≤0.001). These results might indicate a beneficial effect of the application of a SMF for only 1h/day at the short time, which may result prejudicial if applied continuously and for a more extended period of time. Another study has demonstrated that application of a 150 mT SMF on murine MC3T3-E1 osteoblasts induced increased osteocalcin secretion after 15 days 13. Overall, such results suggest important differences between cell types, magnetic field intensity and duration and mode of application, so that individual conditions must be optimized for specific clinical application set-ups.
3.3. No differences in Cellular Distribution or Morphology
In order to determine effects of the SMF on cellular distribution, morphology and cytoskeleton organization, at day 7, we have performed immunofluorescence analysis against phalloidin, a toxin that binds F-actin filaments, and DAPI, a nucleic acid stain commonly used as cell nuclei marker. Overall, we have not observed any differences between osteoblasts cultured as control or exposed to the SMF. Cells were nicely distributed throughout the cell culture well (Fig. 3A,B), connections between the cells remained unaltered and the typical osteoblastic morphology was not lost (Fig. 3C,D). The distribution of the actin fibrils on the cell cytoplasm was normal (Fig. 4). A previous study instead has shown that MG-63 cells treated with 400 mT SMF had more differentiated morphologic features already after 3 days 16, while a SMF of a mere 6 mT has been shown to influence cell membrane integrity 17. Our morphological results instead are coherent with the LDH assay results, which measure membrane disruption, in showing an absence of SMF effects on cell membrane integrity.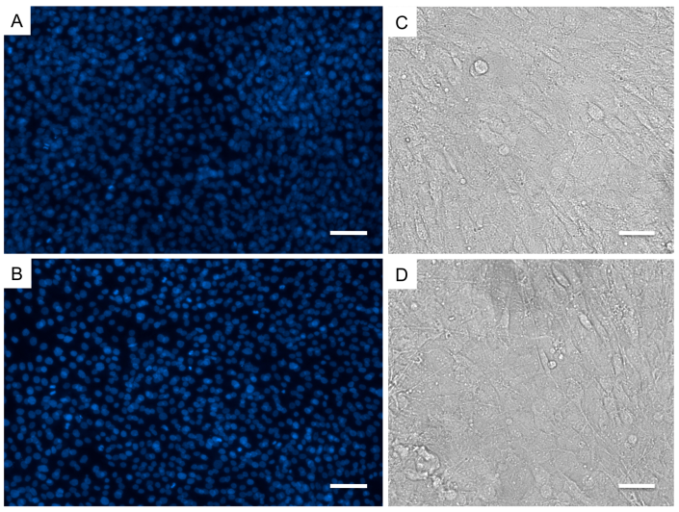 | Figure 3. Analysis of cell distribution and morphology. MG-63 osteoblast-like cell culture under control conditions (A,C) and continuously exposed to a static magnetic field (B,D), at day 7. DAPI staining in blue (A,B) shows no difference in cellular distribution and nuclei integrity. High magnification brightfield imaging (C,D) shows typical osteoblast morphology and intercellular connections. Scale bars: A,B) 100 µm, C,D) 50 µm |
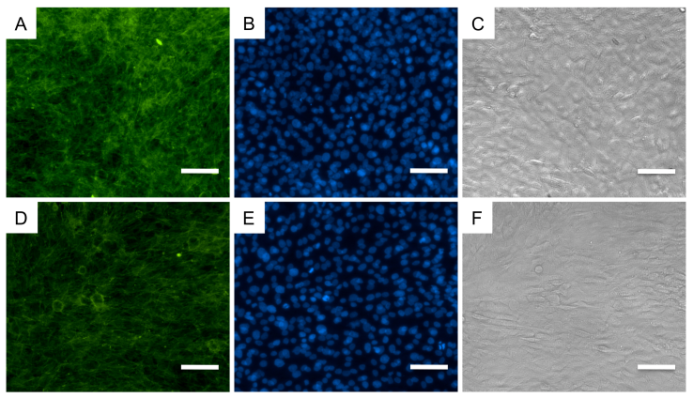 | Figure 4. Actin immunofluorescence analysis. Detailed analysis of cytoplasm matrix organization was performed at day 7 by immunofluorescence staining against phalloidin, a marker of actin filaments (A,D, in green), and respective DAPI staining (B,E, in blue) and brightfield imaging (C,F). A,B,C refer to the control condition, D,E,F refer to continuous exposure to a static magnetic field. Scale bars: 100 µm |
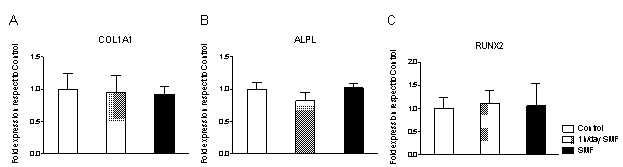 | Figure 5. Gene expression analysis. Relative quantification of gene expression after 7 days of MG-63 culture under the application of a continuous static magnetic field (SMF) or under the application of a SMF for 1h per day, respect to the control, after normalization to the GAPDH reference. A) Collagen type I, alpha 1 (COL1A1), B) Alkaline Phosphatase (ALPL), C) Runt-related transcription factor 2 (RUNX2) |
3.4. No Alteration in COL1A1, ALPL or RUNX2 Gene Expression
Gene expression analysis was performed by reverse transcription quantitative real time PCR, at day 7, for groups control, SMF and 1h/day SMF. Collagen type I, alpha 1 (COL1A1), alkaline phosphatase (ALPL) and Runt-related transcription factor 2 (RUNX2) were analysed, using GAPDH as reference. Collagen type I is the main extracellular matrix component of osteoblast cultures, alkaline phosphatase is a marker of osteoblast activity and Runx2 is associated with osteoblastic differentiation. No difference was observed in their relative gene expression for any of these proteins. Instead, a previous study demonstrated that a low intensity SMF has resulted in decreased ALPL and COL1A1 gene expression on osteoblast cell cultures for up to 14 days 18.
4. Conclusions
This study analysed the effects of a static magnetic field intensity of 320 mT on MG-63 human osteoblast-like cells in vitro. Our results suggest a detrimental effect on cell proliferation by the application of a SMF, either continuously or applied for 1h/day. This reduction was not associated to an increase in cellular apoptosis, stress or disruption of membrane integrity, quantified by the cellular stress marker Lactate Dehydrogenase. Quantification of osteocalcin release, a marker for bone formation, demonstrated that application of a SMF for 1h/day increases osteocalcin release at day 3, while this effect was reversed after 7 days. Instead, the continuous SMF application resulted in decreased osteocalcin release, which might indicate a beneficial effect of the application of a SMF for only 1h/day at the short time, which may result prejudicial if applied continuously and for a more extended period of time. No differences were seen by immunofluorescence analysis in cellular distribution, morphology or cytoskeletonorganization after 7 days in culture. Quantitative PCR analysis for COL1A1, ALPL and RUNX2, all markers of osteoblast activity, identified no alterations in gene expression introduced by the SMF to the cell culture. For sure the effects of magnetic fields on in vitro cell culture depend on cell type, magnetic field intensity and duration and mode of application. This study gives a contribution to understand moderate strength static magnetic field effects on human tissue.
ACKNOWLEDGEMENTS
The authors acknowledge the financial support from European Union project MAGISTERNMP3-LA-2008-214685.
References
[1] | Russo A, Shelyakova T, Casino D, Lopomo N, Strazzari A, Ortolani A, Visani A, Dediu V, Marcacci M. A new approach to scaffold fixation by magnetic forces: Application to large osteochondral defects. Med Eng Phys 2012. |
[2] | Amirfazli A. Nanomedicine: magnetic nanoparticles hit the target. Nat Nanotechnol 2007;2(8):467-8. |
[3] | Arruebo M, Fernandez Pacheco R, Ibarra MR, Santamaria J. Magnetic Nanoparticles for Drug Delivery. Nano Today 2007;2:22-32. |
[4] | Foy SP, Manthe RL, Foy ST, Dimitrijevic S, Krishnamurthy N, Labhasetwar V. Optical imaging and magnetic field targeting of magnetic nanoparticles in tumors. ACS Nano 2010;4(9):5217-24. |
[5] | Jain TK, Reddy MK, Morales MA, Leslie-Pelecky DL, Labhasetwar V. Biodistribution, clearance, and biocompatibility of iron oxide magnetic nanoparticles in rats. Mol Pharm 2008;5(2):316-27. |
[6] | Prijic S, Scancar J, Romih R, Cemazar M, Bregar VB, Znidarsic A, Sersa G. Increased cellular uptake of biocompatible superparamagnetic iron oxide nanoparticles into malignant cells by an external magnetic field. J Membr Biol 2010;236(1):167-79. |
[7] | Panseri S, Cunha C, D'Alessandro T, Sandri M, Giavaresi G, Marcacci M, Hung CT, Tampieri A. Intrinsically superparamagnetic Fe-hydroxyapatite nanoparticles positively influence osteoblast-like cell behaviour. J Nanobiotechnology 2012;10(1):32. |
[8] | Banobre-Lopez M, Pineiro-Redondo Y, De Santis R, Gloria A, Ambrosio L, Tampieri A, Dediu V, Rivas J. Poly (caprolactone) based magnetic scaffolds for bone tissue engineering. Journal of Applied Physics 2011;109(7). |
[9] | Panseri S, Cunha C, D'Alessandro T, Sandri M, Russo A, Giavaresi G, Marcacci M, Hung CT, Tampieri A. Magnetic hydroxyapatite bone substitutes to enhance tissue regeneration: evaluation in vitro using osteoblast-like cells and in vivo in a bone defect. PLoS One 2012;7(6):e38710. |
[10] | Bock N, Riminucci A, Dionigi C, Russo A, Tampieri A, Landi E, Goranov VA, Marcacci M, Dediu V. A novel route in bone tissue engineering: magnetic biomimetic scaffolds. Acta Biomater 2010;6(3):786-96. |
[11] | Panseri S, Russo A, Giavaresi G, Sartori M, Veronesi F, Fini M, Salter DM, Ortolani A, Strazzari A, Visani A and others. Innovative magnetic scaffolds for orthopedic tissue engineering. J Biomed Mater Res A 2012. |
[12] | Tampieri A, Landi E, Valentini F, Sandri M, D'Alessandro T, Dediu V, Marcacci M. A conceptually new type of bio-hybrid scaffold for bone regeneration. Nanotechnology 2011;22(1):015104. |
[13] | Ba X, Hadjiargyrou M, DiMasi E, Meng Y, Simon M, Tan Z, Rafailovich MH. The role of moderate static magnetic fields on biomineralization of osteoblasts on sulfonated polystyrene films. Biomaterials 2011;32(31):7831-8. |
[14] | Wang Z, Che PL, Du J, Ha B, Yarema KJ. Static magnetic field exposure reproduces cellular effects of the Parkinson's disease drug candidate ZM241385. PLoS One 2010;5(11): e13883. |
[15] | Feng SW, Lo YJ, Chang WJ, Lin CT, Lee SY, Abiko Y, Huang HM. Static magnetic field exposure promotes differentiation of osteoblastic cells grown on the surface of a poly-L-lactide substrate. Med Biol Eng Comput 2010; 48(8): 793-8. |
[16] | Huang HM, Lee SY, Yao WC, Lin CT, Yeh CY. Static magnetic fields up-regulate osteoblast maturity by affecting local differentiation factors. Clin Orthop Relat Res 2006;447: 201-8. |
[17] | Dini L, Abbro L. Bioeffects of moderate-intensity static magnetic fields on cell cultures. Micron 2005;36(3):195-217. |
[18] | Denaro V, Cittadini A, Barnaba SA, Ruzzini L, Denaro L, Rettino A, De Paola B, Papapietro N, Sgambato A. Static electromagnetic fields generated by corrosion currents inhibit human osteoblast differentiation. Spine (Phila Pa 1976) 2008;33(9):955-9. |