Sherdil Khan 1, Tiago Cassol Severo 2, 3, Marcos Jose Leite Santos 4, Sérgio Ribeiro Teixeira 1, 3
1Instituto de Fisica, Universidade Federal do Rio Grande do Sul, Av. Bento Goncalves, POA-RS, Brazil
2Centro de Ciências Exatas da Natureza e de Universidade de Caxias do Sul (UCS-CARVI), Bento Gonçalves, RS, Brazil
3Universidade Federal do Rio Grande do Sul (UFRGS), L3FNano, Programa de Pós-Graduação em Microeletrônica (PGMICRO)
4Instituto de Quimica, Universidade Federal do Rio Grande do Sul, POA-RS, Brazil
Correspondence to: Tiago Cassol Severo , Centro de Ciências Exatas da Natureza e de Universidade de Caxias do Sul (UCS-CARVI), Bento Gonçalves, RS, Brazil.
Email: |  |
Copyright © 2016 Scientific & Academic Publishing. All Rights Reserved.
This work is licensed under the Creative Commons Attribution International License (CC BY).
http://creativecommons.org/licenses/by/4.0/

Abstract
Nanotubes of Ta3N5 were synthesized by thermal Nitridation of Ta2O5 nanotubes under ammonia gas environment. The effect of Nitridation temperature and time was evaluated. Electrochemical impedance spectroscopy was applied to study the charge transfer resistance across the Ta3N5 NTs/electrolyte interface. It was observed, at higher temperatures tubular morphology can be preserved for short nitridation time. The electrochemical impedance spectroscopy (EIS) revealed that nitridation conditions such as temperature and time strongly influence the interfacial charge transportation; affecting the photoelectrochemical (PEC) activities of the photoanodes.
Keywords:
Photoelectrolysis, Ta3N5 nanotubes, Photoanode
Cite this paper: Sherdil Khan , Tiago Cassol Severo , Marcos Jose Leite Santos , Sérgio Ribeiro Teixeira , Effect of Thermal Calcinations on the Charge Transfer Resistance of Tantalum Nitride Nanotubes, Nanoscience and Nanotechnology, Vol. 6 No. 1A, 2016, pp. 25-28. doi: 10.5923/c.nn.201601.04.
1. Introduction
In recent years, tantalum nitride (Ta3N5) has received an increasing attention as a photoanode for hydrogen production via photoelectrochemical water splitting under visible light irradiation. [1, 2] Its band structure is near optimal for over all water splitting as it fulfils the straddling of water redox potentials in the band gap. [3] The use of Ta3N5 for hydrogen production via water splitting is relatively new compared to other semiconductors such as Ta2O5, hematite, TiO2 and further investigations are required to better understand its physicochemical properties and to improve its photocatalytic performance. [4-6] Nanotubes of Ta3N5 are of great potential to improve the photoelectrochemical performance of the electrode mainly due to large active surface area. [7, 8] To synthesize Ta3N5 nanotubes at higher temperatures the precursor nanotubes should be synthesized in a manner that helps increasing their adherence on the substrate along with increasing wall thickness. [2] The electrochemical impedance spectroscopy is an attractive tool to investigate the semiconductor- electrolyte interfaces. [5] The interpretation of experimental results can be modelize by equivalent circuits analogy that helps to understand the electrochemical systems provided that particular arrangement of circuit elements in a network possesses physical meaning. [9, 10] Therefore, utilizing electrochemical impedance spectroscopy and equivalent circuits analogy to study the interfacial charge transportation properties of Ta3N5 NTs synthesized under different thermal calcinations conditions are interesting to optimize the photoanode for improved photoelectrochemical activity.In this work, a detailed investigation on the effect of a systematic thermal calcination on the interfacial charge transportation resistance for Ta3N5 nanotubular photoanode is presented.Ta3N5 nanotubes were prepared starting from Ta2O5 nanotubes obtained by anodization. The anodization was carried out in a homemade two electrode sono-electrochemical setup using a Cu disk as cathode and Ta sheet (Alfa Aesar, purity 99.95%) as anode. The anodization electrolyte was a mixed solution of H2SO4 (Lab-Synth Products Laboratory LTD, 98.0%) + 1 vol% of HF and 4 vol% of distilled water. A DC voltage of 50V was applied for 20 min by an initial ramping of 10V/s at 10°C of the electrolyte temperature. To synthesize Ta3N5 as-anodized Ta2O5 nanotubes were placed on an alumina boat and inserted into a horizontal quartz tube oven. The heating and cooling rate of the oven was kept at 5°C/min under a constant 100ml/min gas flux consisting of a special mixture of ammonia: argon (1: 9 vol/vol).For comparison three sets of samples have been synthesized: (a) Constant temperature nitridations at 800°C for 1, 2, 3, 5 and 10 hours.(b) Nitridation at 650°C, 700°C, 750°C, 800°C and 900°C for long time of 10 hours.(c) Nitridation at 800°C, 850°C, 900°C and 1000°C for shorter time of 3 hours.For simplicity the samples were labelled as T°C-time, e.g. the sample nitrided at 650°C during 10h was labelled as 650°C-10h.X-ray diffraction (XRD) patterns from the Ta3N5 nanotubes were obtained by using Siemens, D500 diffractometer with Cu Kα radiation (λ = 1.54 Å) at a 2θ range from 10° to 70° with a 0.05° step size and measuring time of 5 s per step. Rietveld refinements were performed using the same methodology as presented in our previous report. [1] [2] Scanning electron microscopy (SEM) was performed using EVO 50 from Zeiss operating at 10 kV. Electrochemical impedance spectroscopy measurements were performed using an Auto-lab (AUT 84503) potentiostat. The experiments were performed in a quartz cell using standard three-electrode setup employing Ta3N5 nanotubes as working electrode, Pt wobbling as counter electrode and Ag/AgCl as a reference electrode. The electrolyte was 0.1 M K4 [Fe (CN)6 (Merck) and 0.1 mM K3 [Fe (CN)6] (Merck) at pH 7.5. Prior to each measurement the electrolyte was purged by argon gas and photoanodes were cleaned by successive cyclic voltammetry scans. The impedance spectroscopy measurements were performed at a frequency range of 100 kHz – 100 MHz with amplitude of 5 mV applying 0.2 V vs Ag/AgCl.
2. Results and Discussion
Figure 1 displays the morphology of the sample prepared at 800°C for 5 h. It can be seen that the morphology of the nanotubes is sustained due to optimized anodization process used for preparing the starting material i.e. Ta2O5 nanotubes. [2] The as anodized Ta2O5 nanotubes were grown to a length of approximately 1.60 ± 0.03 μm with an average outer diameter and wall thickness of 130 ± 4 nm and 34 ± 2 nm, respectively. [2] However, after the calcinations under ammonia environment the dimensions of the nanotubes decreased. In addition, the grey coloured Ta2O5 sample turned red indicating the phase transition to Ta3N5 nanotubes. Furthermore, the walls of the nanotubes are rough. The features on the walls of the NTs and changes in the geometrical dimensions are related to the density difference between the starting material, Ta2O5 NTs, and the final material, Ta3N5 NTs. [11, 12]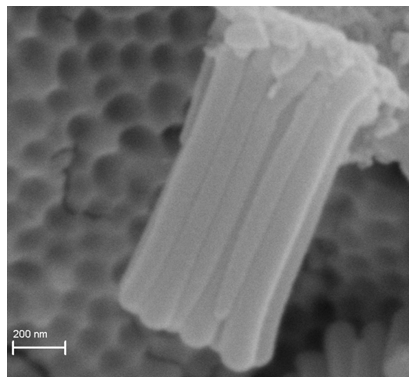 | Figure 1. Ta3N5 nanotubes obtained at 800°C for 5 hours |
Those samples for which the morphology was not sustained were 900°C-10h and 1000°C-3h; however, for rest of all samples prepared in this study the morphologies were tubular. From the Uv-Vis analyses (not shown) the indirect band gap of Ta3N5 nanotubes was found to be ca. 2.1 eV that was red shifted from 3.8 eV of as-anodized Ta2O5 nanotubes that further indicates the phase transformation from Ta2O5 to Ta3N5 nanotubes.The samples were studied by XRD and Rietveld refinements (not shown). From the XRD analyses it was observed that there are certain thermal conditions at which pristine Ta3N5 nanotubes can be synthesized. The obtained Ta3N5 nanotubes presented orthorhombic (JCPDS# 79-1533) phase. It was observed that the Ta3N5 nanotubes can be synthesized as minimum as 650°C; however, long time calcinations were required for the complete transformation to Ta3N5. In addition, sample consisted of amorphism and higher content of oxygen in the crystalline structure as well as in the tubular matrix. On the other hand, at higher temperatures such as 800° or above short time was required to obtain pristine Ta3N5 nanotubes. From Rietveld refinements, it was found that the thermal calcinations under ammonia environment to synthesize Ta3N5 nanotubes triggers the Schottky defects of Ta and N in the lattice along with oxygen substitutional defects. From the occupation factor of the Rietveld refinements the nominal stoichiometries were calculated. It was observed that longer time nitridation of 10h even at 800°C could not result in an improved stoichiometry. These results show that the conventionally used 800°C temperature for the synthesis of Ta3N5 is not an ideal thermal calcinations condition to obtain Ta3N5. However, when time was shortened and temperature was raised to 900°C the chemical composition was closed to the ideal stoichiometry of Ta3N5. For electrochemical impedance spectroscopy the samples were tested in aqueous solution of 0.1 M K4 [Fe (CN)6] and 0.1 mM K3 [Fe (CN)6]. Figure 2 contrasts the Nyquist plots of some selected samples. A semicircle curve can be observed, that confirms a single time constant behaviour of the system under study. Fitting the data according to a single time constant circuit with a resistor in series (Rs) along with the parallel combination of a resistor (Rct) and CPE, as shown in the inset of Fig.2a, we have obtained the charge transfer resistance (Rct) of the samples and presented in Figure 2b. It can be seen that Rct varies with thermal calcinations. The variation of charge transfer resistance confirms a dependency on the nitridation temperature that is related to the different crystalline structure obtained at these conditions. Therefore, the semiconductor/electrolyte interface for each thermal treatment is strongly influenced by thermal calcinations. Comparing the set of samples at fixed temperature of 800°C by varying times, the 800°C-5h has resulted into the lowest Rct (Fig. 2a). On the other hand, due to incomplete nitridation i.e. phase impurities, 800°C-1h has shown highest value of the Rct. For the set of samples prepared for 10h (not shown), 750°C-10h has presented lowest Rct. On the other hand, for 650°C-10h the Rct is highest due to amorphism. In addition, for 800°C-10h, the curve was not completely semicircle; these results strongly suggest that the electrochemical reaction on the surface of such a sample would not be uniform. Therefore, longer time nitridation for synthesizing Ta3N5 NTs either at lower temperature or at higher temperature is not a good choice.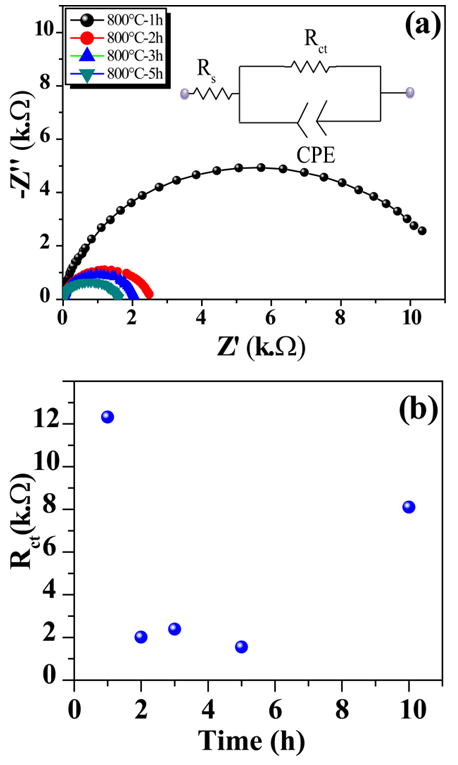 | Figure 2. Nyquist plots of Ta3N5 NTs prepared at different thermal calcinations conditions |
Finally, for the third set of samples, the sample 900°C-3h offers the lowest charge transfer resistance. It was the sample that presented a stoichiometry closer to Ta3N5. These results show that thermal calcinations highly influence the semiconductor/electrolyte interface for Ta3N5 NTs system. Therefore, applying Ta3N5 NTs in photoelectrochemical devices the samples should be synthesized at relatively higher temperature but short nitridation temperature.
3. Conclusions
Ta3N5 nanotubes were prepared at different conditions of thermal calcinations under ammonia environment. It was observed that thermal calcination as a function of temperature and time influence the crystalline structure of Ta3N5 nanotubes. The charge transfer resistance across the Ta3N5 nanotubes/electrolyte interface strongly depends on the calcinations conditions such as temperature and time which is related to the crystalline structure obtained at these conditions. It was observed that long time thermal treatments to produce Ta3N5 nanotubes present structural as well as morphological collapse resulting in to highest charge transfer resistance across the interface. Therefore, Ta3N5 nanotubes should be prepared at higher temperature but short times.
ACKNOWLEDGEMENTS
The authors are grateful to Conselho Nacional de Desenvolvimento Cientifico e Tecnologico (CNPq), Brazil, for the financial support.
References
[1] | Khan S, Zapata M J M, Pereira M B, Goncalves R V, Strizik L, Dupont J, Santos M J L and Teixeira S R Structural, Optical and Photoelectrochemical Characterizations of Monoclinic Ta3N5 Thin Films Phys. Chem. Chem. Phys, 2015. |
[2] | Khan S, Zapata M J M, Baptista D L, Gonçalves R V, Fernandes J A, Dupont J, Santos M J L and Teixeira S R Effect of Oxygen Content on the Photoelectrochemical Activity of Crystallographically Preferential Oriented Porous Ta3N5 Nanotubes The Journal of Physical Chemistry C, 2015. |
[3] | Chun W J, Ishikawa A, Fujisawa H, Takata T, Kondo J N, Hara M, Kawai M, Matsumoto Y and Domen K Conduction and valence band positions of Ta2O5, TaON, and Ta3N5 by UPS and electrochemical methods Journal of Physical Chemistry B 107 1798-803, 2003. |
[4] | Goncalves R V, Migowski P, Wender H, Feil A F, Zapata M J M, Khan S, Bernardi F, Azevedo G M and Teixeira S R On the crystallization of Ta2O5 nanotubes: structural and local atomic properties investigated by EXAFS and XRD Crystengcomm 16 797-804, 2014. |
[5] | Klahr B, Gimenez S, Fabregat-Santiago F, Bisquert J and Hamann T W Photoelectrochemical and Impedance Spectroscopic Investigation of Water Oxidation with “Co–Pi”-Coated Hematite Electrodes J Am Chem Soc 134 16693-700, 2012. |
[6] | Fernandes J A, Migowski P, Fabrim Z, Feil A F, Rosa G, Khan S, Machado G J, Fichtner P F P, Teixeira S R, Santos M J L and Dupont J TiO2 nanotubes sensitized with CdSe via RF magnetron sputtering for photoelectrochemical applications under visible light irradiation Phys. Chem. Chem. Phys. 9148-53, 2014. |
[7] | Cong Y Q, Park H S, Wang S J, Dang H X, Fan F R F, Mullins C B and Bard A J Synthesis of Ta3N5 Nanotube Arrays Modified with Electrocatalysts for Photoelectrochemical Water Oxidation Journal of Physical Chemistry C 116 14541-50, 2012. |
[8] | Zhang P, Wang T, Zhang J, Chang X and Gong J Bridging the transport pathway of charge carriers in a Ta3N5 nanotube array photoanode for solar water splitting Nanoscale 7 13153-8, 2015. |
[9] | Patel D B, Chauhan K R and Mukhopadhyay I Revealing the charge transport mechanism of a photoelectrochemical cell: analysis using A.C. voltage perturbation Phys. Chem. Chem. Phys. 16 20900-8, 2014. |
[10] | Klahr B, Gimenez S, Fabregat-Santiago F, Bisquert J and Hamann T W Electrochemical and photoelectrochemical investigation of water oxidation with hematite electrodes Energ Environ Sci 5 7626-36, 2012. |
[11] | Brauer G, Weidlein J and Strahle J UBER DAS TANTALNITRID TA3N5 UND DAS TANTALOXIDNITRID TAON Zeitschrift Fur Anorganische Und Allgemeine Chemie 348 298, 1966. |
[12] | Zhang Q H and Gao L Ta3N5 nanoparticles with enhanced photocatalytic efficiency under visible light irradiation Langmuir 20 9821-7, 2004. |